A study published in 2003 comparing the nutrition and genetics of broilers from 1957 to a commercially available broiler strain estimated that within less than 50 years of development, broilers were reared to maturation in a third of the time (Havenstein et al., 2003). This shows clear evidence that improvements in genetics, nutrition and management of rearing meat producing chickens has continually improved the growth rate of birds. However, the effects of rapid growth rate on immune function and nutrient requirements of the immune system has not been well defined.
To date requirement studies define the amino acid requirement of the broiler, based on growth and feed efficiency curves in clean experimental conditions. A growing body of research suggests that this definition of requirement may be too narrow, as the requirement for specific amino acids in terms of optimum growth and immune function may be above those required for growth alone (Rama Rao et al., 2003; Abdukalykova et al., 2008; Jahanian, 2009; Ruiz-Feria and Abdukalykova, 2009; Jahanian and Khalifeh-Gholi, 2017; Sigolo et al., 2017). Therefore, under field conditions where environmental stress and pathogen exposure can challenge broilers during the growth cycle, the current requirements may underestimate the real needs for proper immune development and response to these challenges. As impaired health status due to disease or stress can reduce the ability of a broiler to grow at its genetic potential, it is important for us to know how to feed for optimum growth as well as immune function in order to prevent or quickly ameliorate performance losses when such challenges occur.
While management and hygiene practices can and should be utilized to prevent disease, it is not possible to prevent all encounters with pathogenic organisms. To best prevent detriments to growth performance associated with disease, it is advisable to ensure the animal has a strong, fast and adaptable immune system that can efficiently and swiftly manage threats. This will reduce the time in which nutrients may be diverted to the immune system and away from growth. However, in order to raise birds with a highly functioning immune system we need to understand the requirements of the immune system. It is essential to identify which nutrients and the levels of those nutrients that are required for the development of the immune system and to support response to vaccines. Equally important, is to identify if nutrient requirements change when an animal undergoes a disease challenge so we know as a last resort how we can assist the birds to best cope with minimum impact on growth. Finally, it is advisable that we understand the requirements for the arms of the immune system that deal with regulation and can ameliorate inflammation after recovery from an illness or prevent inflammation from being induced by harmless organisms or feedstuffs. Identifying the essential nutrients for all of these diverse functions of the immune system is no small task and to date there is no single solution or easy answer.
In order to redefine nutrient requirements to incorporate the needs for muscle deposition, immune development and immune response, we should understand how these nutrients are utilized by the various systems. The role of amino acids as the building blocks of the proteins comprising muscle, is clear. There are also many studies defining amino acid requirements for muscle deposition and how different amino acids may regulate metabolic processes. This review aims to summarize what is known about how the immune system utilizes specific essential amino acids, in order to predict how amino acid requirements for these amino acids may be redefined when optimal immune function is additionally considered. The literature describing how over- or under-supplementation of the reviewed amino acids affects immune function in broilers will also be summarized, in order to investigate how current feed formulation might be affecting the ability of the birds to maintain their health status and perform to their genetic optimum when challenged.
Interactions between the Immune System and Metabolism
To understand how nutrient recommendations may change if both muscle deposition and immune function are included in the equations that define requirements, the interactions between metabolism and immunity need to investigated. It may not be as simple as increasing all amino acid levels in feed formulations in order to provide sufficient quantities for maintenance of growth and health. Additionally, the effects of immune system activation on metabolism demonstrate the importance of maintaining broiler health in order to optimize performance and subsequently cost efficient production.
It has been well described that acute activation of the immune system often results in the initiation of inflammatory pathways that reduce feed intake (Figure 1). This is described by the hypothalamic-pituitary-adrenal axis and occurs when the immune system recognizes a foreign agent and produces chemical messengers known as cytokines. These cytokines then initiate acute phase protein (APP) production in the liver. Together these cytokines and APPs send feedback to the brain that results in common signs we associate with illness, such as fever and decreased appetite (Bazhan and Zelena, 2013). This feedback mechanism is best studied in mammals (most immune studies are first conducted in mouse models and then confirmed in humans when possible) and while not all of the specific molecules are identical in poultry the same phenomenon has been observed where feed intake is significantly reduced in broilers undergoing a challenge (Iseri and Klasing, 2013; Rochell et al., 2016).
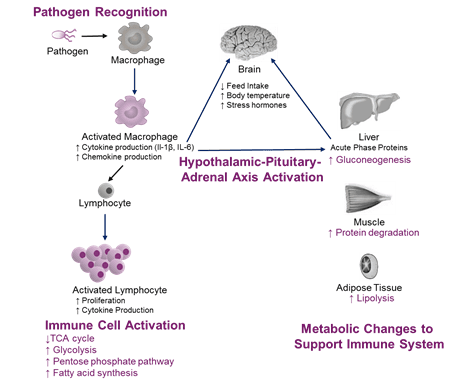
Figure 1. The metabolic pathways involved in a primary immune response to a bacterial pathogen in chickens. Upon recognition of a pathogen macrophages become activated to produce cytokines, this in turn activates lymphocyte populations and signals to the liver to produce acute phase proteins. The cytokine and acute phase protein milieu activate the hypothalamic-pituitary-adrenal axis which may reduce feed intake and induce fever. Metabolic pathway prioritization within immune activated immune cells changes to meet the needs of cytokine production and proliferation, while global metabolic changes provide substrates required for immune function. Adapted from (O'Neill et al., 2016; Htoo, 2018).
Not only is feed intake reduced when a chicken is exposed to a disease, but additionally the efficient utilization of that feed for growth is affected. There are several reasons for this, the most obvious of which may be that if the animal does not have sufficient nutrients to support both growth and immune function it will divert nutrients away from growth towards immunity to survive the challenge. Despite intensive breeding to produce genetic stock that seems to prioritize growth over anything else, it is still an evolutionary advantage to maintain life before any other process.
So far the interactions described have been related to how the immune system affects global metabolism in an animal. An understanding of how the metabolism within individual immune cells changes when they are activated is also useful in determining why nutrient requirements might be altered when an animal is undergoing an immune challenge. In a resting state many immune cell subsets have been found to utilize the tricarboxylic acid (TCA) cycle coupled to oxidative phosphorylation by the electron transport chain, as a very efficient metabolic pathway for providing adenosine triphosphate (ATP), the main energy source for these cells (O'Neill et al., 2016). When immune cells are activated for example through the recognition of a pathogen associated marker, they need to very quickly proliferate and additionally begin to produce cytokines, antibodies, reactive oxygen species etc. For this, they require more than just an energy source like ATP. Proliferating immune cells need intermediates for nucleotide, lipid and protein synthesis. Therefore, activated immune cells will switch their preference towards other cycles like glycolysis or the pentose phosphate pathway that are much less efficient at producing ATP, but are quicker at producing ATP, can be anaerobic and therefore oxygen independent, and are often necessary for the production of substrates needed for cell replication and cytokine production (O'Neill et al., 2016). Upon clearance of an infection the immune system will create regulatory immune cells to suppress the active immune cell populations and memory cell to ensure a rapid response in case of reinfection. Both regulatory and memory immune cells will prioritize the very ATP efficient TCA and oxidative phosphorylation pathways. In the case of memory cells, reactivation by the same pathogen in a second infection will cause them to become activated and switch again to the glycolysis and pentose phosphate pathways utilization for intermediate production.
When the broiler immune system is stimulated by a pathogenic organism the efficiency of feed conversion into protein deposition is significantly impacted (Remus et al., 2014). Even in cases where the animals are diagnosed and treated with antibiotics, this negative impact on feed conversion is still observable (Remus et al., 2014). This is likely due to the fact that by the time birds can be diagnosed the hypothalamic-pituitary-adrenal axis has already been activated. Likewise, the immune cells have already been activated and shifted their biochemical processes. Therefore, relying solely on diagnosis and treatment of broiler flocks already experiencing disease is not the most cost efficient way of dealing with the impacts of immune system stimulation. Management practices to reduce pathogen exposure should be prioritized. However, feed formulations that take into account the needs of immune system to swiftly and efficiently clear infections, will also help to prevent infection and ameliorate the negative effects that immune system stimulation can have on growth.
The remainder of this review will focus on describing key essential amino acids and the immune-relevant biochemical pathways they are involved in, in order to predict the involvement for these amino acids when the immune system is activated. Additionally, literature regarding the impact of deficiencies or increased supplementation of these amino acids in broiler diets and the effect on immune function is summarized. The aim is to make the link between the hypothesized effects of these amino acids on specific immune pathways and the changes to amino acid requirements observed when the broiler chicken’s immune system is activated.
Methionine Requirements for the Immune System
Polyamine synthesis
Polyamines are required within individual cells for many important processes involved in rapid cell proliferation, including transcription, mRNA stabilization and translation. Polyamines can be vital for proper viability of cells (membrane stability and DNA stabilization), as well as cell functions including cell-receptor binding (Peranzoni et al., 2007; Bjelakovic et al., 2010; Minois et al., 2011). Methionine is an essential precursor for the synthesis of polyamines; putrescine, spermidine and spermine (Figure 2). The synthesis of these polyamines is generally upregulated in inflammation; however, so is the catabolism of these polyamines (Minois et al., 2011) . Additionally, polyamines have been found to play an integral role in both the induction and regulation of inflammation. Polyamines were shown to be essential to immune responses to pathogen associated molecular patterns (Puntambekar et al., 2011) and specifically spermine has been implicated in many instances of regulation of inflammatory processes (Bjelakovic et al., 2010). Therefore, limiting factors to polyamine synthesis such as deficiency in the precursor amino acids methionine and arginine can be hypothesized to impair acute inflammatory responses as well as regulation of these responses.
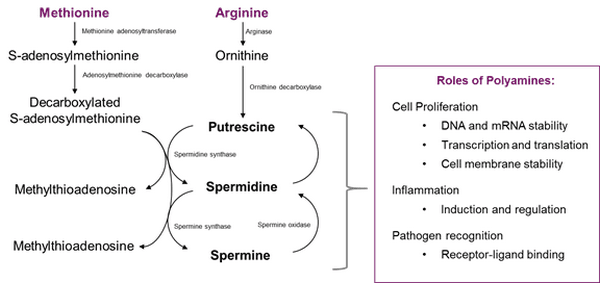
Figure 2. The role of methionine and arginine in the synthesis of polyamines (putrescine, spermidine and spermine) and the various roles of polyamines in immune function. Adapted from Gallus gallus specific pathways on KEGG PATHWAY database ‘Cysteine and methionine metabolism’ and ‘Arginine and proline metabolism’ (Kanehisa, 2002).
Oxidative Stress
Glutathione in a reduced state is an important anti-oxidant as it acts as an electron acceptor for free radicals. Cysteine is a precursor for this antioxidant which can be supported through the degradation of methionine in the homocysteine pathway (Figure 3). Therefore, the availability of these sulphur amino acids (SAA), methionine and cysteine, may be increasingly important in situations where oxidative stress occurs. Oxidative stress may occur is in the initiation of an innate immune response. The production of reactive oxygen species, like nitric oxide, is utilized by innate cells like granulocytes and macrophages to kill ingested pathogens (Singh et al., 2012; Brune et al., 2013; Iseri and Klasing, 2013). These free radicals that are produced to kill pathogens must be regulated so that they do not induce oxidative stress that can impact healthy host cells; glutathione production can play a role in maintaining the redox balance within these innate immune cells that produce reactive oxygen species (Droge et al., 1998; Morris et al., 2013; Hughes et al., 2017; Martinez et al., 2017).
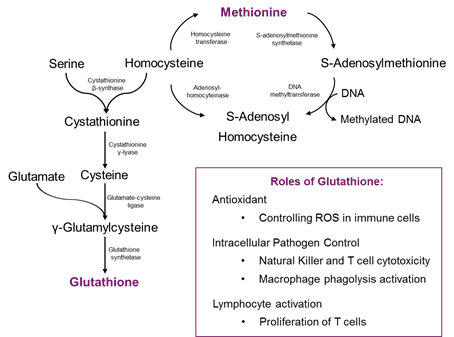
Figure 3. The role of methionine in the synthesis of glutathione and the various roles of glutathione in immune function. Adapted from Gallus gallus specific pathways on KEGG PATHWAY database ‘Cysteine and methionine metabolism’ and ‘Glutathione Metabolism’ (Kanehisa, 2002).
Control of intracellular infections
Glutathione’s role in immune response goes beyond regulating oxidative stress. Glutathione is also involved in several signalling pathways utilized for the activation, proliferation and effector functions of immune cells specifically involved in controlling intracellular infections. When a pathogen infects a host cell it can be difficult for many immune cells and components like antibodies, to find the intracellular pathogen in order to fight it. Natural killer cells of the innate immune system and cytotoxic T cells of the adaptive system are specialized in finding pathogens, like viruses, that may be hiding within infected host cells (Saremi, 2016; Whelan, 2018). When an infected cell is found these natural killer cells and cytotoxic T cells either send a message through a receptor-ligand interaction that instructs the cell to self-destruct, or they create a pore in the cell to transfer lethal enzymes into the infected cell to kill it. This ensures the infection can’t spread and that healthy neighboring cells are uninfected. The killing mechanisms described have been found to be severely impaired when the reduced form of glutathione is low (Droge and Breitkreutz, 2000; Morris et al., 2013). The reduced form of glutathione has also been shown to improve macrophage activation by helper T cells for better control of pathogens within these innate cells (Droge and Breitkreutz, 2000; Morris et al., 2013). The activation and proliferation of T helper cells, who help coordinate immune responses to specific pathogens, is likewise impaired when cells are deficient in glutathione (Droge et al., 1998; Droge and Breitkreutz, 2000). Therefore, it can be postulated that infections involving intracellular pathogens, like many viral challenges, may increase the SAA requirement in the diet to provide substrates for glutathione synthesis needed to support the specialized immune cells involved in those responses.
Regulation of gene expression
Immune cells are produced through a process known as hematopoiesis, which is initiated when stem cells in the bone marrow first differentiate into either myeloid progenitors that give rise to most of the innate immune cells (like monocytes, dendritic cells, and granulocytes), or lymphoid progenitors which give rise to natural killer cells and the adaptive immune cells (B cells and T cells). The process of producing these progenitor cells heavily relies on methylation of the genes being expressed, to stabilize them during the hematopoietic process (Suarez-Alvarez et al., 2012). DNA methylation of promotor regions (the gene region responsible for turning the expression of that gene on or off) is mostly associated with suppression of gene expression. Therefore, active demethylation of promotor genes in the progenitor cells begins to turn on the genes in these cells that differentiate them from progenitors into the specific cell types they will become (Suarez-Alvarez et al., 2012). Methionine is a vital component of the homocysteine pathway that leads to the creation of methyl-donors for DNA methylation (Figure 3). Therefore, a deficiency in dietary methionine may lead to a deficiency in methyl donors and an impairment of the development and differentiation of immune cell populations.
Methionine requirements for immune function in broiler chickens
The role of methionine in various immune pathways has been compiled from research conducted in many different species, mostly mammalian. Therefore, it is important to also review the role of methionine in immune development and response specifically of chickens in order to validate whether there really is an increased dietary requirement for methionine when the broilers immune system responds to a challenge.
Several studies have shown that the dietary requirement for SAAs for growth may not be meeting the requirement for immune function. Tsiagbe et al. (1987) conducted a series of trials to compare the growth performance and immune response of broilers with diets including different SAA levels in the diet. They found that optimal performance for growth was reached at a lower dose than was observed for optimization of immune function, as measured by total antibody and T cell proliferation response (Tsiagbe et al., 1987). Another study confirmed the importance of SAA for T cell proliferation in broilers, as spleen lymphocytes from broilers fed SAA deficient diets had impaired responses to a T cell specific stimulant (Konashi et al., 2000). In 2003, it was also confirmed by another group that SAA requirements continued to significantly improve total antibody titers at several inclusion levels above where growth performance effects plateaued (Rama Rao et al., 2003). Additional dietary SAA above the broiler requirements for growth may also support innate immunity. In a study comparing four different genetic lines of broilers with various methionine levels it was determined that while the lowest SAA level in the diet was already sufficient for optimum growth, that innate immune reactions continued to increase in a dose dependent manner to 3 higher supplementation rates of methionine (Rama Rao et al., 2003).
The improvement in immune cell numbers and activation with additional methionine has also been correlated to improved responses to pathogen associated markers, suggesting improved resistance to poultry relevant pathogens. A study was conducted to look at the effects of SAA supplementation on the response to a commonly utilized immune stimulation model where birds are injected with a cell wall component, lipopolysaccharide (LPS), from pathogenic Eschericia coli (Takahashi et al., 1997). Birds fed a SAA deficient diet had an inhibited immune response to the challenge as observed by a decreased plasma levels of APPs and inflammatory cytokines, compared to the treatment group fed sufficient SAA diets (Takahashi et al., 1997).
These studies collectively indicate that if SAA requirements for broilers are calculated based on performance values alone that immune function may not be optimized. In scenarios where intensive vaccination strategies are planned, or risk of infection is greater, it could be beneficial to increase dietary methionine above the current Evonik or breeder recommended requirements in order to assist immune function without detriments to performance.
Arginine Requirements for the Immune System
Immune cell production
Immune cell progenitors all arise from hematopoietic stem cells in the bone marrow. The progenitor cells that will become T cells move from the bone marrow to the thymus to finish their training, while the progenitor cells that will become B cells move to the Bursa of Fabricus (a specialized organ in avian species) to complete their maturation. Arginine has been found to act as a secretagogue, stimulating the release of hormones, like growth hormone, from the pituitary gland in the brain (Adriao et al., 2004; Olinto et al., 2012; Cordoba-Chacon et al., 2013). Growth hormone in turn stimulates the production of hematopoietic stem cells in the bone marrow, increasing the number of immune cells entering the blood stream (Koo et al., 2001; Adriao et al., 2004; Weigent, 2013). This arginine dependent release of hormones has also been linked to an increase in the migration of naive immune cells to the thymus, maintenance of the thymus and differentiation of T cells (Adriao et al., 2004; Weigent, 2013; Savino et al., 2016). Therefore, an arginine deficiency may also lead to a deficiency in the production and maturation of various immune cell populations and therefore impair immune function.
Innate immune cell functions
Macrophages are innate immune cells best known for their ability to eat relatively large components like live bacterial pathogens or dead cell debris through a process known as phagocytosis. However, these cells actually have several phenotypes that support diverse functions. The differentiation of macrophages into specific phenotypes is largely dependent on the way they utilize arginine (Figure 4). Macrophages involved in inflammatory responses that need to engulf live bacteria are known as classically activated macrophages. These classically activated macrophages utilize arginine to produce a reactive oxygen species known as nitric oxide, which is important for killing the engulfed pathogens (Peranzoni et al., 2007; Muraille et al., 2014). Alternatively activated macrophages describe a phenotype involved in some parasite infections, wound healing and regulation of T cell populations. These macrophages utilize arginine to synthesize ornithine, which can be utilized for production of polyamines that were described in the chapter on methionine (Peranzoni et al., 2007; Muraille et al., 2014). Ornithine is also highly important for collagen deposition during wound healing (Peranzoni et al., 2007; Muraille et al., 2014).
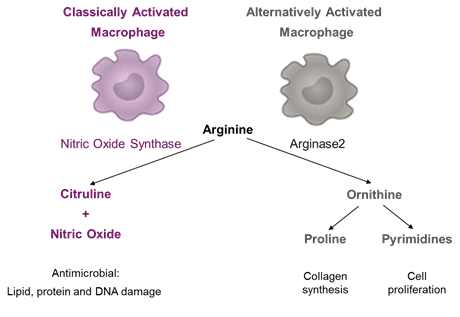
Figure 4. The role of arginine in the function of classically activated macrophages for antimicrobial activity and of alternatively activated macrophage for wound healing mechanisms. Adapted from (Muraille et al., 2014) with Gallus gallus specific pathways from KEGG PATHWAY database ‘Arginine and proline metabolism’ (Kanehisa, 2002).
Adaptive immune cell functions
T cells are members of the adaptive immune system. Like macrophages, they have diverse functions depending on their phenotype including the coordination of anti-bacterial inflammation, initiation of anti-parasitic and allergic responses, active killing of virus infected cells, stimulation of B cell antibody production and the regulation of all of these active responses. One thing that all of these diverse T cell subsets have in common, is the surface expression of a very specific T cell receptor. Without this surface receptor a T cell is unable to be activated, proliferate and perform its role (Peranzoni et al., 2007). Arginine has been shown to be very important in both maintaining the viability of T cell receptors, in regulating proliferation of T cells after activation and in the long term survival of memory T cell subsets (Peranzoni et al., 2007; Geiger et al., 2016).
The importance of arginine to broiler immune function
The role of arginine in immune development and function has been specifically studied for broiler chickens and confirms the hypothesized importance of the amino acid in immune function based on multi-species literature reviews and broiler specific pathway analysis. Additional dietary arginine above growth requirements has been observed to improve spleen and bursa development, increase populations of both innate and adaptive immune cells, and improve antibody responses (Abdukalykova et al., 2008; Jahanian, 2009; Ruiz-Feria, 2009; D'Amato and Humphrey, 2010; Jahanian and Khalifeh-Gholi, 2017). Additional dietary arginine was also found to to increase the expression of amino acid transporters in immune cells present in the blood and thymus (D'Amato and Humphrey, 2010). This suggests that arginine is not only involved in the production of immature immune cells, but in the targeted uptake of amino acids when these cells become active effector cells.
Several broiler-specific studies have also looked at the effect that dietary arginine has on the response to the immune stimulant LPS. In these studies dietary arginine was found to be important for the maintenance of immune cell populations, for macrophage production of nitric oxide, for regulating inflammatory cytokines and for maintaining body weight through the challenge (Takahashi et al., 1999; Kwak et al., 2001; Tan et al., 2014; Lieboldt et al., 2017).
The observed improvement in immune organ development, immune cell numbers and responses to stimulants suggests that dietary arginine is important for protective responses, thereby improving broiler resistance to specific diseases. There are several infection studies supporting this hypothesis. In an Eimeria acervulina infection model, it was reported that broilers fed high levels of arginine had higher production of nitric oxide prior to, but not during the infection (Rochell et al., 2017). Despite the lack of difference in immune response during the acute phase of infection in this study, increased dietary arginine did significantly improve growth efficiency in the challenged birds. This suggests that the available arginine was prioritized for immune function over growth, as increased dietary arginine did not affect resistance to this specific disease, but did prevent the reduced growth performance resulting from increased requirements of the immune system. This was confirmed in another Eimeria spp. challenge trial that observed no effect of dietary arginine levels on the expression of most inflammatory genes investigated, but did report an improvement in feed intake and feed efficiency of challenged broilers when higher levels of arginine were included in the diets (Tan et al., 2014).
Eimeria spp. infections in poultry can also be a predisposing risk factor for the development of a bacterial pathogen, Clostridium perfringens, which causes Necrotic Enteritis (Timbermont et al., 2011). In an Eimeria spp. and C. perfringens co-infection challenge model, additional dietary arginine was able to ameliorate intestinal damage from the disease model, increase both inflammatory and regulatory cytokine expression and reduce the abundance of C. perfringens in the intestines (Zhang et al., 2017a). The reduction in the bacterial pathogen populations was hypothesized to be due to the production of nitric oxide from arginine in macrophages, as a bacterial pathogen killing mechanism. Conversely, in another bacterial challenge using a Salmonella enterica Typhimurium infection model, additional dietary arginine with vitamin E was able to increase the activity of innate immune cells in the broiler, but not to decrease Salmonella spp. counts in the ceca (Liu et al., 2014). Unlike the parasite challenge studies, these bacterial pathogen challenge models suggest that additional dietary arginine may directly assist in anti-bacterial immune responses.
Arginine has also been reported to be very important in the immune response to viral diseases of poultry. Increasing dietary arginine in layers challenged with infectious bronchitis was shown to increase the numbers of innate immune cells in both the blood and respiratory tract (Lee et al., 2002). Cytotoxic T cells, which are specialists in finding and killing virus infected cells, were also increased in the lungs from infected birds when fed higher arginine levels (Lee et al., 2002). Infectious bursal disease virus vaccines are often found to impair immune function as they maintain a certain amount of virulence in order to be protective. In studies investigating the effects of infectious bursal disease vaccine it was uniformly reported that broilers receiving higher doses of arginine, had higher post-vaccine antibody titers as well as increased cytotoxicity and proliferation of T cells; all of which culminated in increased vaccine protection (Tayade et al., 2006; Ruiz-Feria and Abdukalykova, 2009; Emadi et al., 2011; Bulbul et al., 2014; Tan et al., 2015; Jahanian and Khalifeh-Gholi, 2017). Similar results were obtained in a study investigating the effects of arginine supplementation in broiler diets prior to vaccination with a hydropericardium syndrome virus vaccine, as higher arginine diets improved the efficacy of the vaccine improving growth, survival and reduction of virus (Munir et al., 2009). One potential mechanism by which increased dietary arginine may benefit anti-viral immune responses is through the increase in substrate for nitric oxide production in macrophages. Several studies have found that the production of nitric oxide was able to inhibit replication of Marek’s disease virus as well as a reovirus in chicken macrophages (Pertile et al., 1995; Djeraba et al., 2000; Xing and Schat, 2000). Like the bacterial challenge models, the virus and viral vaccine challenge models indicate that increasing arginine in feed formulation for poultry may improve viral specific immune responses.
The roles of arginine in immune function are diverse and involved in development, activation and maintenance of immune function. Therefore, it is unsurprising that the requirement for arginine is reported to increase in various kinds of immune system challenges of poultry including parasitic, bacterial and viral infections.
Threonine Requirements for the Immune System
Mucin Production
The greatest borders between the inside of the body and potentially harmful agents in the environment, are the mucosa-associated lymphoid tissues. Many of these borders, like the bronchus-associated lymphoid tissue and gut-associated lymphoid tissues, are lined with a layer of thick protective mucus to provide a barrier between microorganisms in the lumen and the epithelial cells lining the lungs or gut. This mucus can help clear parasitic worm infections in the gut by continuously clearing mucus before parasites can attach to epithelia (Johansson and Hansson, 2016). Likewise, the mucus layer provides a physical barrier for bacterial infections as successful enteric bacterial pathogens must develop ways to penetrate mucus with flagella or proteolytic degradation in order to infect epithelial cells (Johansson and Hansson, 2016). In models inducing a lack of specific mucus components animals become highly susceptible to intestinal infections and chronic inflammation, likely due to over-exposure of epithelial cells to pathogens (Bergstrom et al., 2010). Mucus is comprised of mucins, which are glycosylated proteins containing a proline-threonine-serine rich region that can make up 50 % or the protein structure (Johansson and Hansson, 2016). For some secreted mucins, the threonine levels have been estimated as high as 11 % in some species (Gum, 1992). It is therefore logical that restriction of dietary threonine has been shown to inhibit mucin production (Faure et al., 2005).
Environmental triggers like pathogen infections or bacterial fermentation in the gut occurring in the presence of high fiber diets, can stimulate increased mucus production and subsequently threonine requirements (Johansson and Hansson, 2016). For example, in a rat sepsis model of inflammation the threonine requirement was significantly increased in order to support not only mucus production, but also plasma and intestinal wall protein synthesis (Faure et al., 2007). The same group validated their findings in a mini-pig model showing that the uptake of threonine from the gut lumen was increased in pigs with inflamed intestines compared to healthy controls in order to support increased mucus production (Remond et al., 2009). Furthermore, it has been reported that mucins are not degraded by digestive enzymes in the lumen or reabsorbed resulting in a net loss of threonine when mucus is secreted into the gut lumen (Remond et al., 2009). Therefore, conditions like increased pathogenic load or high fiber diets may increase dietary threonine requirements in order to support both mucin expression and protein deposition for muscle growth.
Antibody Production
Antibodies, also known as immunoglobulins, are an important component of the immune system of mammalian and avian species alike. They can neutralize pathogens to prevent them from binding to host cells, signal pathogen killing cascades or increase phagocytic uptake of pathogens by immune cells. Antibodies are produced by specialized immune cells called B lymphocytes. Each B cell, as well as the antibodies it produces, are highly specific to a single protein marker called an antigen. Antigens are often found on the surface of pathogens which allow them to be recognised by the immune system and activate B cells to produce antibodies in response to infection or vaccination (which mimics infection) to help fight and prevent pathogen driven disease.
Basic antibody structure is comprised of two light polypeptide chains bound to two heavy polypeptide chains by disulfide bonds to form a Y like structure (Figure 5). The constant region at the bottom of both chains is more conserved between antibodies and it is this portion of the antibody that will be bound to host immune cells or interact with their receptors. The top of both chains is the portion that binds to antigens and is comprised of what are known as variable regions. The variable regions are aptly named as this region can vary immensely in amino acid structure between individual antibodies. This allows the immune system to combat an exponentially large number of potential pathogens with a very diverse network of these highly specific antibodies. Threonine has been implicated in mammalian studies as being a major component of antibodies and was estimated to be as high as 10 % of milk immunoglobulins (Bowland, 1966). This may be due to the hinge region of antibodies which is rich in serine and threonine (Putnam et al., 1981; van der Boog et al., 2005). Avian antibodies vary a little in structure, specifically in this hinge region; however, it appears that threonine may be equally important in formation of immunoglobulins in these species. The peptide sequences available for the chicken immunoglobulin light chain contain the highest proportion of serine and threonine compared to most other amino acids (The UniProt, 2017). Specific portions of the variable region of antibodies are also largely comprised of small amino acids, like threonine and these regions have been reported to be significantly longer in chicken antibodies compared to mammalian antibodies (Wu et al., 2012). Not only are these regions longer in chickens than mammals, but the proportion of small amino acids in these regions (glycine, serine, alanine, cysteine and threonine) is greater (Wu et al., 2012). Therefore, it can be postulated that around vaccination schedules or during disease challenges, when antibody production is high, an increase in the dietary inclusion level of amino acids like the SAA and threonine could be required to support growth and immunity.
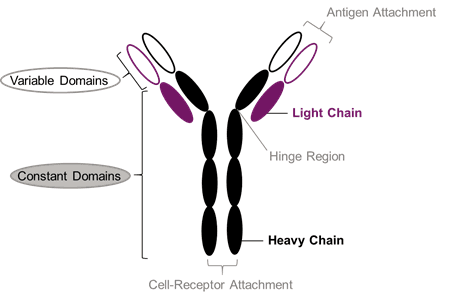
Figure 5. The structure of chicken immunoglobulin Y. Constant region domains are indicated with filled ellipses, while variable region domains (rich in threonine and serine) are indicated with open ellipses. The heavy chain is illustrated in black while the variable chain (rich in small amino acid sequences like threonine) is illustrated in purple. The hinge region as well as the attachment sites for antigens and receptors on host cells are also shown. Adapted from (Conroy et al., 2014).
The importance of threonine to broiler immune function
It has already been reported that requirements for threonine in broilers may be largely dependent on feed ingredients, environment of rearing and pathogen exposure (Kidd and Tillman, 2016). For example, high fiber diets have been shown to increase the threonine requirement, which is postulated to be due to both increased bacterial fermentation in the gut and/or mucus sloughing (Chee et al., 2010; Wils-Plotz and Dilger, 2013). In broilers similar effects have been observed, in which increased threonine was required to improve performance and support increased mucus layer thickness when mannan-oligosaccharide containing diets were fed (Chee et al., 2010). Conversely, when soluble fibers like pectin and cellulose were fed to broilers, the threonine requirement was found to be reduced, but this was concluded to be a reflection of the significantly reduced weight of birds fed the insoluble fiber in this study (Wils-Plotz and Dilger, 2013). Vaccination may also increase the threonine requirement of broilers to support antibody response, as increased threonine above maintenance requirements was shown to improve both non-specific antibody responses, as well as production of antibodies specific to New Castle Disease post-vaccination (Sigolo et al., 2017). The use of built-up litter as opposed to new litter is an example of an environmental factor that has been observed to increase threonine requirements for broilers (Corzo et al., 2007). This is postulated to be the result of increased exposure to various parasitic, bacterial or viral pathogens in the litter, that subsequently stimulate the need for increased immune response including mucus and/or antibody production.
Many pathogenic infections may increase dietary threonine requirements for broilers. For example, coccidiosis in poultry, caused by Eimeria spp. parasites, has been shown to increase mucin gene expression in the intestines and also increase the humoral immune response to the parasite (Mockett and Rose, 1986; Brito Lda et al., 2014). Therefore, additional dietary threonine above maintenance requirements may support Eimeria spp. specific immune responses. A study feeding dietary threonine in excess of an established requirement for unchallenged animals, reported that high dietary threonine improved weight gain, feed intake and feed efficiency in the coccidiosis challenged birds, without affecting Eimeria oocyst counts in the intestines (Wils-Plotz et al., 2013). However, the high threonine diet also improved performance in the unchallenged animals in this trial, so it was not clear if the high threonine level was meeting requirement for controls or challenged birds. It was hypothesized that even higher doses beyond those tested may further improve immune response variables (Wils-Plotz et al., 2013). This theory is supported by studies investigating threonine deficient diets. One such study reported that the effects of coccidiosis challenges were clearly exacerbated in broilers fed threonine deficient diets as these birds had significantly lower weight gain and increased intestinal Eimeria oocyst counts compared to birds fed sufficient threonine diets (Zhang et al., 2016).
An early study appears to confound with the majority of results as it concluded that there was no increased requirement for threonine in a mild single Eimeria spp. infection, based on the lack of interaction effect observed between threonine level and challenge on any growth parameters investigated (Kidd et al., 2003). However, in this study despite three dietary threonine levels being tested it was again unclear if the requirement was determined in either unchallenged or challenged birds as no plateau in growth performance effects was observed for either group (Kidd et al., 2003). It is difficult to conclude from such studies if either a more severe coccidiosis challenge or higher dietary threonine levels would have result in differences in the performance or immune response in challenged animals.
As previously described Eimeria spp. infections are often used as predisposing elements in C. perfringens driven Necrotic Entertis challenge models. In one such Necrotic Enteritis challenge trial the threonine to lysine rations in broiler diets was not shown to affect the incidence or severity of lesions, nor the challenge induced mortality (Star et al., 2012). However, the reduction in weight gain and feed intake resulting from the challenge was significantly improved when the threonine to lysine ration in the diet was increased beyond the requirement established for uninfected birds in the same trial (Star et al., 2012). Similar results were obtained in other bacterial challenge models of broilers. In a Salmonella Enteriditis infection model the level of dietary threonine did not significantly affect the quantity of Salmonella colonies quantified in the intestines or the number of Salmonella positive birds (de Barros Moreira Filho et al., 2015). However, higher threonine was able to ameliorate the negative effects of the Salmonella challenge on weight gain which may have been associated with increased number of intestinal goblet cells and mucin expression in the affected intestinal region (de Barros Moreira Filho et al., 2015).
These studies collectively show that while insufficient dietary threonine may inhibit immune responses to various pathogen infections, it is unclear whether dietary threonine levels above growth requirements may further improve immune responses. There is a consistent body of evidence to support the role of increased threonine in broiler diets for the prevention of growth detriments incurred by the immune response to pathogens, likely because a proportion of dietary threonine is being redirected away from growth to prioritize immune function in these scenarios.
Tryptophan Requirements for the Immune System
Tryptophan as a substrate for serotonin synthesis
The production of serotonin is probably one of the best studied examples of the uses of tryptophan beyond growth performance, especially for broilers. Serotonin is synthesized from tryptophan and is a neurotransmitter involved in gastrointestinal motility, wound healing and mediating stress responses (de Las Casas-Engel and Corbi, 2014; Olivier, 2015; Martin et al., 2017). Increased serotonin production has been linked to reduction in aggressiveness and fear responses (de Boer et al., 2017), which could help reduce the risk of injury during handling or when animals are housed with high stocking density. Stress and acute inflammation also initiate stress hormone release through the hypothalamic-pituitary-adrenal axis (Figure 1) which is negatively correlated to serotonin activity (de Boer et al., 2017), therefore, it could be that higher levels of serotonin, and therefore tryptophan, are required to mediate the stress hormone response to environmental stimuli.
Tryptophan in acute phase protein synthesis
Acute phase proteins are quickly produced by the liver and released into the blood circulation in response to acute inflammation. These APPs can help immediately in the response to specific pathogens by starving them of vital nutrients or triggering their uptake by immune cells (Reeds et al., 1994; Kurpad, 2006). The APPs are also vital in the systemic activation of the immune response (Figure 1). The amino acid composition of APPs is relatively high in the aromatic amino acids (phenylalanine, tyrosine and tryptophan), which is likely why the serum levels of tryptophan and phenylalanine increase in early stages of infection when acute phase proteins are being produced in the liver (Kurpad, 2006). In humans catabolism of muscle protein has been observed to be initiated shortly after injury or infection in order to meet the amino acid requirements for APP production (Reeds et al., 1994). As these proteins are relatively high in the aromatic amino acids (like tryptophan) compared to skeletal muscle the catabolism of muscle proteins may be driven to meet the requirements of these amino acids that are now limiting. This results in a net nitrogen loss as other amino acids, like lysine, are liberated in muscle catabolism, but required in low amounts comparatively in production of APPs (Reeds et al., 1994). Therefore, increasing dietary levels of aromatic amino acids around times of stress or illness may prevent muscle catabolism and the associated impairments to growth and efficiency of protein utilization to support APP synthesis.
Tryptophan as a precursor for important coenzymes
The coenzyme nicotinamide adenosine dinucleotide (NAD) and it’s phosphorylated form (NADP) are required for various biochemical processes within cells, including the production of ATP as an energy source and the repair of DNA (Moffett and Namboodiri, 2003; Badawy, 2017; Grohmann et al., 2017). The redox balance between oxidized (NAD+) and reduced forms (NADH) of NAD is also important for controlling oxidative stress in cells (Moffett and Namboodiri, 2003; Badawy, 2017; Grohmann et al., 2017). In most cells and tissues, niacin (aka vitamin B3) is utilized as the primary substrate for the de novo synthesis of NAD; however, tryptophan can also be utilized for synthesis of NAD (Figure 6). In diets high in tryptophan the excess not utilized for protein synthesis will be converted in the liver (where the majority of tryptophan degradation occurs) to NAD+ or oxidized to produce energy (Badawy, 2017).
Immune cells like monocytes, macrophages, dendritic cells, B cells and T cells specifically utilize tryptophan degradation pathways to store a precursor of NAD production, called quinolinate (Moffett and Namboodiri, 2003). This suggests that in immune cells, tryptophan degradation may be the primary source of NAD coenzyme for immune cells (Moffett and Namboodiri, 2003). During acute phases of infection, innate immune cells produce nitric oxide in order to kill pathogens once the cell ingests them (as described in the arginine chapter). The synthesis of this reactive oxygen species has been shown to require NAD or NADP as a cofactor (Kujundzic and Lowenthal, 2008) and the electron transfer between reduced and oxidized forms has been postulated as a mechanism to replenish homeostasis after the induction of this oxidative stress by these reactive oxygen species in early immune responses (Moffett and Namboodiri, 2003).
The storage of quinolinate by immune cells may also be a mechanism to prepare for the metabolic shift towards glycolysis and fatty acid synthesis pathways that occurs when immune cells are activated and must proliferate (O'Neill et al., 2016), as NADP is a reducing agent utilized in these pathways (Figure 6). This may also explain why tryptophan degradation outside of the liver is comparatively minimal in homeostasis, but significantly increases during immune activation, specifically in lymphoid tissues (Badawy, 2017).
Figure 6. The degradation of tryptophan by indoleamine 2,3-dehydrogenase (IDO) through the kynurenine pathway to produce kynurenines and coenzymes NAD+ and NADP. The roles of kynurenines as well as the coenzymes are described. Adapted from (Moffett and Namboodiri, 2003) and the Gallus gallus specific KEGG PATHWAY database ‘Tryptophan metabolism’ and ‘Nicotinate and nicotinamide metabolism’ pathways (Kanehisa, 2002).
Tryptophan in immune regulation pathways
While activation of the immune system is necessary for defense against pathogens, sustained immune activation and inflammation can be detrimental to the host organism. Therefore, regulatory pathways of the immune system are equally as important as activation pathways in sustaining long term health of an animal. In acute phases of inflammation many immune cells upregulate the expression of indoleamine 2,3-dioxygenase (IDO) which degrades tryptophan through the kynurenine pathway (Figure 6). If inflammatory cytokines are present this enzyme is quickly degraded (Moffett and Namboodiri, 2003; Grohmann et al., 2017). Conversely, if regulatory cytokines are present a positive feedback loop for the expression of this enzyme is initiated (Moffett and Namboodiri, 2003; Grohmann et al., 2017) . This is because the initiation of this kynurenine pathway of tryptophan catabolism plays a major in regulating the immune activity in immune cells. When antigen presenting cells express IDO and begin degrading tryptophan, they take on a regulatory rather than activating phenotype (Lob et al., 2009; Grohmann et al., 2017). In other words, rather than activating immune cells to respond to pathogens, this special subset of dendritic cells interacts with different immune cells to regulate the immune response after the infection has been cleared (Figure 7). For example, these regulating dendritic cells can induce apoptosis of active immune cells or regulate specific functions like decreasing antibody production by B cells, receptor expression by T cells or creating regulatory types of T cells to further control the immune response (Lob et al., 2009; Grohmann et al., 2017; Hippen et al., 2017).
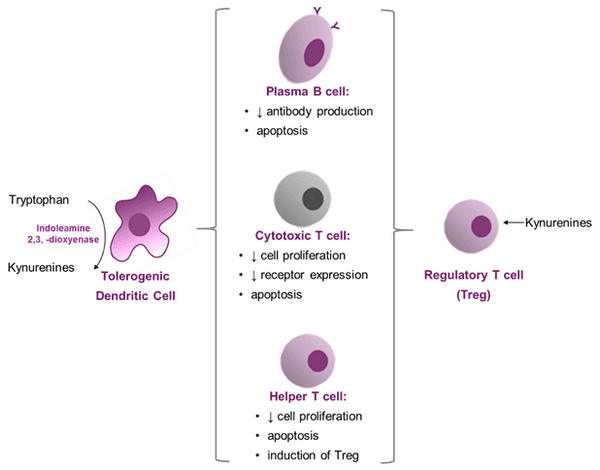
Figure 7. The role of the tryptophan degradation in the induction of tolerogenic dendritic cells and T regulatory cells and their downstream effects on lymphocyte proliferation, activity and apoptosis. Adapted from (Lob et al., 2009)
While tryptophan might be utilized in resting state by immune cells to build up stores of coenzyme precursors and during immune activation for the production of APPs, it is also depleted during the induction of immune regulation at the cessation of an immune response. Therefore, additional tryptophan beyond that required for growth may be required for the development of competent immune cell populations, the activation of innate responses to immune stimulation, and during regulation of inflammation and return to homeostasis.
The importance of tryptophan for broiler immune function
The best studied effects of dietary tryptophan beyond growth for broilers, relates to the production of serotonin which may be because the serotonergic system and its development has been well defined in chickens for several decasdes (Okado et al., 1992). Increasing dietary tryptophan has been observed to increase serotonin, decrease stress related hormones like corticosterone and reduce aggressive behaviour or fear response in chickens (Shea et al., 1990; Mench, 1991; Blair et al., 1993; Newberry and Blair, 1993; Rosebrough, 1996; Shea-Moore et al., 1996; Corzo et al., 2005). For example, increasing dietary tryptophan levels in broiler breeder males was shown to reduce corticosterone levels and aggressive pecking associated with skip-a-day feed restriction, likely due to the increased serotonin production observed (Shea et al., 1990; Mench, 1991; Shea-Moore et al., 1996). Negative effects of handling stress on broiler performance have also been reported to be reduced in response to increasing dietary tryptophan (Newberry and Blair, 1993).
The relationship between available tryptophan and the production of acute phase proteins has not been specifically investigated in avian species, as was previously summarized for humans. However, it has been reported that increased dietary tryptophan in layers reared under heat stress was able to not only improve antioxidant status and antibody response, but also to increase production of APPs (Dong et al., 2012). This suggests that additional dietary tryptophan can support APP synthesis in chickens, at least in this specific scenario. A study looking at the tryptophan requirements of male broilers reported that dietary tryptophan deficiency decreased nitrogen retention, which may be due to the lack of availability for precursors of APPs (Corzo et al., 2005). However, it should be noted that this study was conducted without inducing any immunological challenge so the production of such proteins would have been relatively low and was not directly correlated to the decrease in nitrogen retention.
It is known that the kynurenine pathway at the heart of tryptophan catabolism used for NAD production and immune regulation does exist in chickens (Figure 6). Additionally, cell culture studies using chicken macrophages have observed that tryptophan degradation via the kynurenine pathway is important for the production of nitric oxide in response to pro-inflammatory stimuli (Kujundzic and Lowenthal, 2008). An in vivo study also confirmed that the cytokine and antibody response to an infectious bursal disease virus challenge was improved when excess tryptophan was added to the diet (Emadi et al., 2011). These studies support the role of tryptophan in the induction of immune responses in chickens; however, the role of tryptophan degradation on regulation of immune function (Figure 7) has not been specifically investigated in chickens. One study suggests that tryptophan may play also play a role in immune regulation as increased dietary tryptophan was shown to ameliorate oxidative stress in broilers reared in a high stocking density environment (Wang et al., 2014). This could be hypothesized to be due to the degradation of tryptophan to produce NAD for redox modulation with NAD+/NADH. The role of kynurenines in induction of specific regulatory subsets of immune cells has yet to be reported in chickens; however, the kynurenine pathway may still be a mechanism involved in restoring homeostasis after an immune response.
The Roles of Other Essential and Non-Essential Amino Acids in the Immune System
The biochemical roles of the essential amino acids methionine, arginine, threonine and tryptophan in the immune system and the current findings regarding these amino acids specifically in broiler immune function have been summarized in detail. However, other essential amino acids are also hypothesized to play an important role in immunity based on comparative literature reviews of mammalian species. The branched chain amino acids (BCAAs) have been reported in mammalian studies to be involved in immune function (Konashi et al., 2000; Thornton et al., 2006; Zhang et al., 2017b). For example, leucine is important in pathways initiating lymphocyte cell proliferation and active functions of cytotoxic T cells and natural killer cells (Zhang et al., 2017b). Another BCAA, isoleucine, has been implicated in the production of antimicrobial peptides in the gut, while valine may improve innate immune function. However, BCAA studies in broilers are very limited. One study indicated that BCAA deficiency in broiler diets increased lymphocyte proliferation to a stimulus, which is against proposed theories for the role in immune function based on cross-species literature reviews (Konashi et al., 2000). Another study concluded that dietary valine levels had no effect on the innate or adaptive immune functions in broilers that were measured (Thornton et al., 2006).
Several non-essential amino acids are also commonly found in biochemical pathways that are highly relevant to immune function. Glutathione production in poultry not only requires SAAs, but also involves serine and glutamate as precursors (Figure 4). Glutamine can be utilized for synthesis of glutamate or arginine and can therefore support the immune functions already described for those amino acids. Mucin synthesis also requires relatively high amounts of proline and serine in the proline-threonine-serine rich region discussed in the threonine section (Johansson and Hansson, 2016). Likewise, antibody structure in chickens is not only high in threonine, but also very high in small non-essential amino acids like glycine, serine, alanine and cysteine (Wu et al., 2012). These non-essential amino acids, while relevant to immune function, are not specifically supplemented to poultry diets as they can be synthesized de novo and the requirements are usually met through the dietary crude protein. Therefore, literature reporting the specific role of these non-essential amino acids for broiler immune function or how these requirements might change in challenging conditions is limited. Therefore, as research continues with the aim of reducing dietary crude protein for cost-efficient, sustainable broiler production (Payne, 2007), the impact of crude protein on immune development and function of broilers should also be considered.
Summary
This review comprises what is known about some key essential amino acids in the development of the immune system and the response to external stimuli such as vaccination and pathogen exposure. However, specific recommendations to change amino acid requirements in poultry feed formulations are not provided. This was a purposeful omission for several reasons. The first reason for not defining specific amino acid requirements for immune function is that the studies reporting the effects of varying amino acid supplementation rates were conducted over several decades and included diverse dietary, environmental and genetic variables. This makes the studies difficult to compare when attempting to define a recommendation for the modern broiler strains and conditions that are current industry standard for any given region. The second reason that specific amino acid recommendations are not provided for challenging conditions is due to the diversity of challenges the birds must face. The amino acid needs for immune development, vaccination response, parasite control, bacterial response, anti-viral immunity and immune regulation could vary quite greatly as the cells and pathways involved in these different arms of the immune system are diverse. Additionally, for enteric pathogens the utilization of amino acids in the lumen by the pathogen itself may alter the availability to the animal and intestinal damage could affect digestibility.
The currently available literature provides a thorough understanding of the amino acids important to different cells and pathways within the immune system. However, continued systematic research programs to investigate the amino acid requirement changes for specific poultry relevant diseases and stresses should be conducted with modern broiler strains in order to answer the question if the dietary requirements need to be redefined when risk of those illnesses is high. This should of course not come at the cost of other management and pharmaceutical practices to reduce disease risk, such as biosecurity practices and vaccination, respectively. However, nutritional strategies to support the development of a healthy immune system and the prevention of disease should be considered as an area with great potential to reduce the cost of disease to broiler performance and therefore the broiler producer.
Abbreviations
APPs – acute phase proteins
ATP – adenosine triphosphate
BCAAS – branched chain amino acids
IDO – indoleamine 2,3 dehydrogenase
LPS – lipopolysaccharide
NAD - nicotinamide adenosine dinculeotide
NAD+ – oxidized NAD
NADH – reduced NAD
NADP – phsoprylated NAD)
SAA – sulphur amino acid
TCA - tricarboxylic acid