INTRODUCTION
In poultry, phosphorus (P) is an essential nutrient in metabolic processes and a component of nucleic acids, membrane phospholipids, and bones (Nie et al., 2013). Much effort has been made to increase P absorption in poultry to improve metabolic performance and reduce the environmental impact of phosphate (Pi) release from manure (Ahmadi and Rodehutscord, 2012; Letourneau-Montminy et al., 2013).
Dietary P is absorbed in the small intestine by both passive and active transport processes. Active transport of intestinal Pi is mainly mediated by sodium-dependent Pi transporters that belong to the Solute Carrier 34 (SLC34) family (Murer et al., 2001; Forster et al., 2013). Intestinal Pi absorption and renal Pi resorption are regulated by type II NaPi cotransporters (NaPi-II), which are further subdivided into NaPi-IIa (SLC34A1) and NaPi-IIb (SLC34A2) (Hilfiker et al., 1998; Werner and Kinne, 2001). The NaPi-IIb cotransporter is considered to be the major Na-Pi cotransporter that is expressed in the brush border membrane of the small intestinal epithelium but is also expressed in the lung and secretory tissues (Hilfiker et al., 1998; Feild et al., 1999). In chickens, NaPi cotransporter mRNA abundance was greatest in the duodenum, intermediate in the jejunum, and lowest in the ileum (Huber et al., 2015; Li et al., 2018).
A number of factors regulate expression of Pi transporters. Dietary P is a main modulator of Pi transporter expression (Sabbagh et al., 2011; Huber et al., 2015). Broilers and laying hens fed diets restricted (0.20/0.25% vs. 0.40/0.55% of diet) in nonphytate phosphorus (NPP) increased NaPi-IIb mRNA abundance in all segments of the small intestine (Yan et al., 2007; Nie et al., 2013). The expression of NaPi-IIb mRNA in the duodenum during 1 to 21 D of age decreased linearly (P < 0.002) as dietary NPP levels increased from 0.10 to 0.50% (Liu et al., 2017). This effect was dependent upon both the magnitude and length of dietary Pi deprivation (Capuano et al., 2005; Radanovic et al., 2005). Vitamin D is also part of the control mechanism for Ca and P absorption, because it can influence NaPi-IIb cotransporter gene transcription (Christakos et al., 2014; Han et al., 2018). In the kidney, 2 enzymes regulate vitamin D levels. Vitamin D-1α-hydroxylase catalyzes the conversion of 25-hydroxy vitamin D to the hormonally active 1α, 25 dihydroxy vitamin D, while vitamin D-24-hydroxylase converts the active form of vitamin D into inactive calcitroic acid (Christakos et al., 2010; Shanmugasundaram and Selvaraj, 2012).
Supplementation of minerals as nanoparticles, which are defined as particles less than 100 nm, increases the surface area, which potentially could increase absorption and utilization leading to higher bioavailability and a reduction in the quantity of supplements needed for broiler production (Weiss et al., 2006; Nikonov et al., 2011; Al-Beitawi et al., 2017; Sabry et al., 2018). The P digestibility depends on solubility of the limestone, particle size of the Ca source, in addition to total Ca concentration in the broiler diet (Kim et al., 2018). The bioavailability and effects of nanocalcium phosphate (Nano P) compared with dicalcium phosphate (Dical P) on broiler performance have been studied. Vijayakumar and Balakrishnan (2014) reported that the bioavailability of calcium phosphate nanoparticles was 200% when compared with Dical P. Hassan et al. (2016) similarly showed that a diet containing Nano P at only 25% of NPP levels was equivalent to 100% Dical P and resulted in a 50% reduction in excreted Ca and P. These findings may have great potential in the poultry industry especially in feed management by minimizing mineral loss in fecal material, which is both an economic and environmental issue. The growing concerns with regard to the potential contribution of P in poultry excreta on eutrophication of surface waters has led to increasing pressure to limit excess P in poultry rations, thus reducing fecal output of P and the resulting environmental contamination (Waldroup, 1999). Although nanoparticles are showing promise in animal agriculture, it is important that the safety of nanomaterials to both the animals and the environment, such as soil biota, is carefully considered (Mishra et al., 2017; Sabry et al., 2018).
The objective of the current experiment was to determine the relative bioavailability of dietary Nano P compared with Dical P and the effects of various concentrations of these two P sources on performance and mRNA abundance of duodenal NaPi-IIb and kidney hydroxylases when fed to broiler chickens from 1 to 14 D of age.
MATERIALS AND METHODS
Birds and Management
All animal procedures were approved by the Virginia Tech Institutional Animal Care and Use Committee. A total of 648, day-old unsexed Cobb 500 broiler chicks were obtained from a commercial hatchery in Virginia. Chicks were individually weighed and allocated to 72 cages (9 treatments of 8 replicate groups of 9 chicks) in electrically heated battery brooders (377 cm2/bird), so that bird weights were similar across all pens. Experimental diets were randomly assigned to cages of chicks. Over the 14 D experimental period, the temperature was maintained at 34°C from day 1 to 3 posthatch, 31°C from day 4 to 7, and was progressively reduced to 27°C by day 14. The light cycle was set to 24 h of light for day 0 and 23 h of light for day 1. Lighting was adjusted to 18 h of light from day 2 to 14. The chicks were provided ad libitum access to water and mash feed.
Diets
Nine experimental mash starter diets (Table 1) were formulated based on maize and soybean meal and were formulated to meet nutrient requirements of chickens according to the recommended allowances of the breed (Cobb 500 broiler performance and nutrition supplement guide, 2015) with the exception of available phosphorus (AvP). Two main sources of P were used to design the experimental treatments: Dical P and Nano P. Calcium phosphate nanopowder (American Elements Co., Los Angeles, CA) contained 98.5% calcium phosphate (39.90% Ca and 18.50% P) with a Ca:P ratio of 2.15:1.00. The particle size ranged from 500 to 1,000 μm for Dical P and < 100 nm for Nano P. Experimental diets were formulated to contain 0.20, 0.24, 0.28, 0.32, and 0.36% AvP by adding Dical P or Nano P (Table 1). All diets were formulated to contain the same metabolizable energy (3,035 kcal ME/kg), crude protein (21.51%), and Ca (0.90%). Diets contained 0.25% titanium dioxide as an indigestible marker.
Animal Performance
Bird BW (g/chick) were averaged by cage for statistical analysis. Feed offered was measured on day of hatch (doh) and day 14, while feed refusal was measured on day 7 and day 14. Feed offered and feed refusal were then used to determine feed intake (FI, g/pen) between doh and day 14. Feed intake was calculated for each cage by [(initial feed pan and feed weight + feed added over duration of experiment) – feed pan weight and remaining feed]. Total cage BW gain (final BW at day 14 – initial BW at doh) and FI were used to calculate feed efficiency (FE, g gain per g feed intake). Mortality was recorded daily, and any dead birds were weighed for inclusion into the FE calculation to adjust FE for mortality. Feed efficiency was calculated as total pen weight gain plus mortality weight gain divided by pen feed intake.
Apparent Ileal Phosphorus Digestibility
On day 14, all remaining birds were euthanized by cervical dislocation. The small intestine was immediately removed, and the ileal contents of each bird between Meckel’s diverticulum and the ileal-cecal junction were collected by gentle manipulation for determination of apparent ileal phosphorus digestibility (AIPD). Owing to the small amount of intestinal contents, all samples within a replicate cage were pooled together for analysis resulting in 9 replicate samples per treatment as outlined by Douglas et al. (2003). Ileal contents were dried at 55°C for 72 h and then ground using a mill grinder to pass through a 0.50 mm sieve and stored frozen at −20°C until laboratory analysis. Once ground, both feed and ileal samples were analyzed to determine P and Ti concentrations. Feed samples were analyzed in quadruplet, while ileal samples were analyzed in duplicate. One g of feed or 0.5 g of dried ileal digesta was weighed into a 125 mL Erlenmeyer flask. Twenty mL of sulfuric acid and 2 mL of nitric acid were then added to each flask. Samples were boiled for 5 min, then allowed to cool completely. Another 2.5 mL of nitric acid were added to each flask and boiled for an additional 20 min before being cooled completely to form a clear colored solution free of precipitates. The last step was repeated with 1.0 mL of nitric acid if the solution was not colorless and not free of precipitates. The digested sample was then standardized using a 100 mL volumetric flask by addition of sample and deionized water to exactly 100 mL. The standardized samples were then poured through circular 90 mm filter paper, and 50 mL samples were retained in 50 mL conical tubes for final analysis (Boguhn et al., 2009). Samples were sent to the Virginia Tech Soil Testing Laboratory for an inductively coupled plasma atomic emission spectroscopy analysis (ICP) to determine P and Ti concentrations. The ICP measures characteristic atomic emission spectra. Samples were introduced into a nebulizer to create an aerosol, which in turn were introduced into argon plasma. In the plasma, at approximately 8,000 K, molecules were almost completely dissociated, and the resulting ions emitted absorbed energy as a characteristic wavelength of light. The light intensity emitted at each wavelength was proportional to the amount of the element that was excited. A calibration curve was created so that the unknown samples could be measured against known standards. Determined values were represented as mg/L (Martin et al., 1994). Total digesta and feed P and Ti values were determined by multiplying the weight of the original sample by the dilution factor and the final P and Ti concentrations in solution. The following equation was used to determine AIPD:

where (P diet) is the phosphorus determined concentration in the diet; (P ileum) is the concentration of phosphorus in the ileal digesta; (Ti diet) is the determined concentration of titanium in the diet; and (Ti ileum) is the determined concentration of titanium in the ileal digesta.
Tissue Sampling
All birds euthanized for digesta collection on day 14 also had the right leg collected to determine tibia ash weight (TAW) and tibia ash percentage (TAP). Tibias from the right leg were removed, packaged in labeled plastic bags, and frozen at −20°C. Collected legs were autoclaved at 121°C at 18 psi for 30 min, and all adhering tissue was removed including both tibia cartilage caps. Bones were then dried in a convection oven at 100°C for 24 h (Persia et al., 2003). The dried bones were weighed before they were dry-ashed for 24 h at 600°C in a muffle furnace. The bones from all 9 birds per replicate pen were pooled to generate a single sample for analysis (n = 8). The remaining ash was weighed to determine tibia ash, expressed as total ash g per tibia and as a percentage of dry bone. Six euthanized birds per treatment were randomly selected for collecting intestinal samples. The duodenal segment (from distal of the gizzard to 1 cm distal of the bile duct) was isolated immediately from the gastrointestinal tract (Rodehutscord et al., 2012). The gut lumen was opened at the mesenterial border and gently rinsed with ice-cold phosphate buffered saline (1%) to remove gut contents. Intestinal tissues were scraped with a glass microscope slide to collect the mucosa from the underlying muscle layer from a 5 cm section in the center of the duodenum, frozen in liquid nitrogen, and stored at −80°C. Kidney samples were also collected from these birds. Kidney tissue was minced and then placed immediately into RNAlater solution (RNAlater, Ambion, Austin, TX) and stored at −80°C until RNA extraction.
RNA Extraction, Reverse Transcription, and Real-Time qPCR
Total RNA was extracted from the intestine and kidney samples (n = 6 per treatment) with Trizol reagent and Direct-zol RNA MiniPrep kit (Zymo Research Inc., Irvine, CA) according to the manufacturer’s instructions. The concentration and purity of the RNA were determined by measuring the absorbance at 260 and 280 nm. After extraction, total RNA was reverse transcribed into cDNA by using 2.0 μL of 10xRT Buffer, 0.8 μL of 25xdNTP Mix, 2.0 μL of 10xRT Random Primers, 1.0 μL of MultiScribe Reverse transcriptase (Applied Biosystems, Foster City, CA), 10 μL of RNA (0.2 mg/mL), and 4.2 μL of RNase-free water. The mRNA abundances of duodenal NaPi-IIb, kidney vitamin D-1α-hydroxylase, and vitamin D-24- hydroxylase mRNA, and the reference gene β-actin were determined by real-time quantitative PCR, using the primers listed in Table 2. A 10 μL reaction was prepared containing 5 μL of fast SYBR Green Master mix (Applied Biosystems), 1.0 μL of forward primer (5 μM), 1.0 μL of reverse primer (5 μM), 1.0 μL of cDNA (diluted 1:30), and 2.0 μL of RNase-free water. The PCR reaction was conducted in a 7,500 Fast RealTime PCR instrument (Applied Biosystems). The amplification protocol used was 95°C for 20 s followed by 40 cycles of 95°C for 3 s and 60°C for 30 s. Each sample was amplified in triplicate and amplification efficiency was determined by standard curves to ensure equal efficiency between target genes and the reference gene. Fold change was calculated using the 2−ΔΔCT method of Schmittgen and Livak (2008).
Statistical Analysis
Data were analyzed using PROC GLM, version 9.2 (SAS Institute, 2004). For BW, FI, TAW, and TAP data, a slope regression line for various concentrations of P from Dical P and Nano P was calculated. The analysis of covariance test was used to compare the slope regression lines for Dical P and Nano P (Onyiah, 2008). For FE and AIPD data, a factorial analysis (2 P sources and 4 AvP levels) was used. For gene expression data, one-way ANOVA was used to compare the various concentrations of P from Dical P and Nano P treatments vs. control. When significant differences were noted, Tukey’s Honestly Significant Difference test was used to separate means, and significance was accepted at P ≤ 0.05.
RESULTS AND DISCUSSION
This experiment investigated the relative bioavailability of dietary Nano P compared with Dical P, and the effects of various concentrations of these 2 P sources on performance and mRNA abundance of intestinal NaPi-IIb and kidney hydroxylases when fed to broiler chickens from day 1 to 14. Regression analysis performed for both BW (Figure 1A) and FI (Figure 1B) for Dical P and Nano P fed at various concentrations showed significant positive linear responses to supplemental P from both sources. For both BW (P = 0.75) and FI (P = 0.71), there were no differences between the slopes for Dical P and Nano P. The response for FE (Figure 2A) showed a P source x P level interaction. At 0.28% AvP, FE for Nano P was lower than Dical P; whereas at all other AvP levels, there was no difference between Nano P and Dical P.
The increased BW gain and FI with increased dietary AvP in the current experiment were similar to Jiang et al. (2013) who reported that increasing concentrations of NPP (0.25, 0.35, and 0.45%) in starter broiler diets increased (P ≤ 0.01) both BW (560.5, 662.1, and 670.5 g) and FI (52.88, 61.93, and 62.38 g/d), respectively. These responses are expected as dietary concentrations of AvP or NPP were generally below the requirement of starter chicks for performance allowing for generation of a linear slope response to estimate bioavailability (NRC, 1994; Ammerman et al., 1995). The negative impact of P deficiency on BW gain, FI, and FE was in birds fed the 0.20% NPP diet (574 g/ bird, 821 g/bird, and 698g:kg, respectively) compared with those fed 0.40% NPP diet (798 g/bird, 984 g/ bird, and 813 g:kg, respectively) from days 6 to 22 (Babatunde et al., 2019). The similar slopes between the Dical P and Nano P fed chicks, and the differences in growth response between previous reports and the current data suggest that there is minimal to no difference in P bioavailability between Dical P and Nano P supplementation. Our result is in contrast to those reported by Hassan et al. (2016) and Vijayakumar and Balakrishnan (2014) who reported that Nano P had increased bioavailability compared with Dical P. The difference in results could be because of the method of analysis and levels of P used. In our study, we used slope regression of BW, FI, TAW, and TAP to assess bioavailability, while Hassan et al. (2016) used BW, FI, and FCR, and Vijayakumar and Balakrishnan (2014) used BW and tibial bone ash, calcium, and phosphorus content to assess bioavailability. In addition, in our study, total P ranged from 0.46 to 0.64%; whereas, Hassan et al. (2016) used NPP levels from 0.21 to 0.45%.
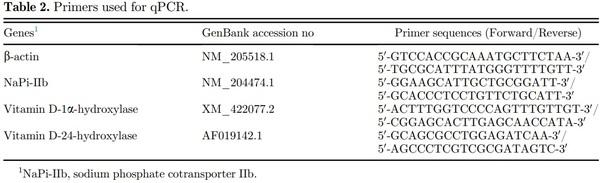
Regression analysis was performed for both TAW (Figure 1C) and TAP (Figure 1D) for Dical P and Nano P fed at various concentrations, resulting in significant positive linear responses to supplemental P from both sources. For both TAW (P = 0.40) and TAP (P = 0.83), there were no differences between the slopes generated for Dical P and Nano P responses. The slope regression analysis generated slope value responses that were similar to those of the BW gain and FI, providing further support that Dical P and Nano P had similar bioavailability. It is of note that the fit of the regression lines was more consistent with bone mineral analysis in comparison to direct performance responses.
These results were not surprising and were in agreement with previous reports (Powell et al., 2011; Shastak et al., 2012; Li et al., 2015; Gautier et al., 2018). Broiler birds that received a low-NPP diet (0.20%) showed reduced TAP (33.7%; P < 0.01)compared with birds fed the 0.40% NPP diet (48.4%) from days 6 to 22 (Babatunde et al., 2019). This is because of the importance of P and Ca as components of hydroxyapatite in the bone and because 80% of P found in the body is located in the bone. Thus, an insufficient dietary P level in low P diets led to adverse effects on bone formation and mineralization except in the presence of endogenous enzymes such as phytase (Olukosi et al., 2013).
Apparent ileal P digestibility % (Figure 2B) showed a P source x P level interaction. At 0.24% AvP, AIPD for Dical P was greater than Nano P; whereas, at all other levels of AvP (0.28–0.36%), AIPD was not different between Dical P and Nano P. Furthermore, AIPD at 0.24% AvP from Dical P was greater than AIPD at all other levels of AvP regardless of P source, with the exception of 0.28% Dical P. These data are in contrast to Hamdi et al. (2015), where increasing NPP concentrations of 0.30, 0.35, 0.40, and 0.45% increased AIPD 76.8, 81.3, 82.3, and 86.1%, respectively (P ≤ 0.01). These differences demonstrate that mineral digestion is a complex process that depends on a number of physiological variables, including but not limited to transporter abundance and vitamin D metabolism. Babatunde et al. (2019) showed that there was a decrease (P < 0.01) in AIPD in birds fed a 0.20% NPP diet as compared with birds fed a 0.40% NPP diet from days 6 to 22. Tibia ash may reflect a cumulative process and may not react as quickly to changes in dietary P as observed in the ileal digestibility of P.
The Ca:P ratio can affect P digestibility. Liu et al. (2013) reported greater digestibility when birds were fed a diet with a Ca:total P ratio of 0.8; whereas, there was no difference in P digestibility for Ca:total P ratios between 1.2 and 2.0. Because the Ca:total P ratios ranged from 1.4 to 2.0 in our study, we did not expect any differences. As expected, Nano P showed no difference in AIPD for 0.24 to 0.36% AvP; however, AIPD for Dical P at 0.24% AvP was greater than that at 0.32 and 0.36% AvP.
In the duodenal mucosa, mRNA abundance of the NaPi-IIb transporter was greatest in the 0.20% AvP fed birds compared with all other concentrations of P for both Dical P and Nano P (Figure 3A). There was no difference, however, in mRNA abundance between 0.24, 0.28, 0.32, and 0.36% P for both Dical P and Nano P. Consistent with our results, Li et al. (2016) showed no difference in NaPi-IIb mRNA abundance when broilers were fed both low and high nutrient density diets supplemented with 0.25 to 0.4% NPP. The inverse relationship between NaPi-IIb mRNA abundance and dietary P levels has been reported previously for both broilers and layers. Yan et al. (2007) were the first to clone and functionally characterize chicken NaPi-IIb. They found that Na Pi-IIb mRNA was expressed greatest in the duodenum followed by the jejunum and ileum and that a reduction of P in the diets of Ross 308 broilers from hatch to day 4 posthatch led to an induction of Na-Pi-IIb mRNA abundance. Nie et al. (2013) also showed a similar decrease in duodenal Na-Pi-IIb mRNA abundance, when Dwarf pink shell laying hens were fed increasing levels of NPP from 28 to 41 wk of age.
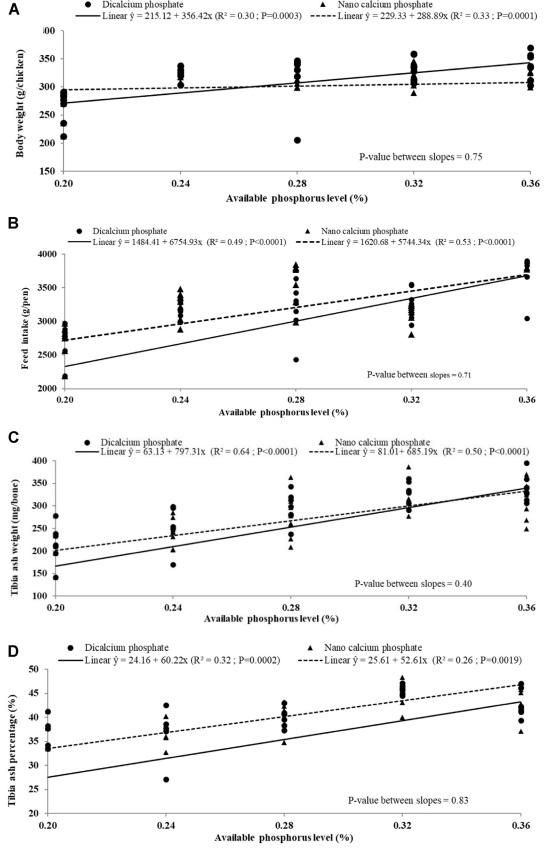
Figure 1. Body weight (A), feed intake (B), tibia ash weight (C), and tibia ash percentage (D) of chicks fed diets containing 0.20, 0.24, 0.28, 0.32, and 0.36% available phosphorus from either dicalcium phosphate (circles and solid lines) or nanocalcium phosphate (triangles and dashed lines) from 0 to 14 D. Slope regression analysis was performed to determine differences in response slopes between the 2 sources of P for body weight, feed intake, tibia ash weight, and tibia ash percentage.
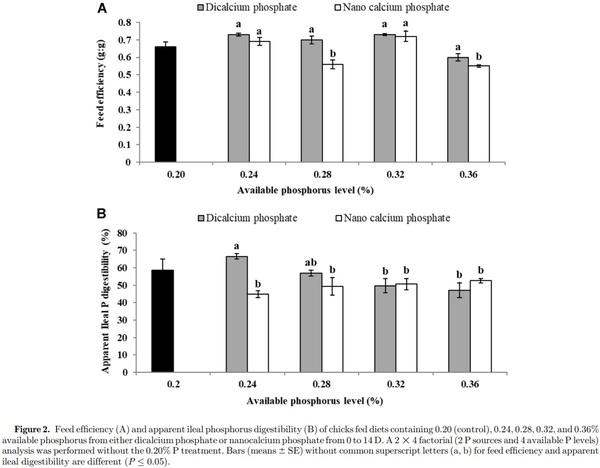
The NaPi-IIb gene expression is regulated by dietary P and crude protein levels in broiler chickens (Xue et al., 2016; Liu et al., 2017). Using Western blots, Huber et al. (2015) detected 2 isoforms of NaPi-IIb protein of 105 kDa and 90 kDa in the jejunum but only the 90 kDa protein in the ileum. Supplementation of the diet with monocalcium phosphate did not affect expression of either NaPi-IIb isoform. Phytase supplementation, however, resulted in decreased jejunal expression of the 90 kDa NaPi-IIb protein. A comprehensive analysis of the effects of high and low Pi diets on not only NaPi-IIb mRNA and protein abundance but also Pi activity was conducted by Fang et al. (2012). In this study, Ross broilers fed a 0.6% total Pi diet, had maximal Pi uptake in the jejunum even though the greatest NaPi-IIb mRNA and protein abundance was in the duodenum. Furthermore, when 40-day-old broilers were fed low (0.2% Pi), normal (0.6% Pi), or high (1.0% Pi) diets for 7 D, there was increased NaPi-IIb mRNA and protein abundance and Pi uptake in the duodenum of the low Pi group, but no difference in the jejunum and ileum. Li et al. (2018) showed that diets fed to laying hens containing increasing levels of AvP (0.15–0.82%) caused an increase in NaPi-IIb protein but not NaPi-IIb mRNA in the duodenum and a decrease in NaPi-IIb mRNA but not NaPi-IIb protein in the ileum. These results demonstrate that regulation of NaPi-IIb mRNA and protein varies with intestinal segment and that Pi uptake and NaPi-IIb mRNA and protein levels are not always correlated.
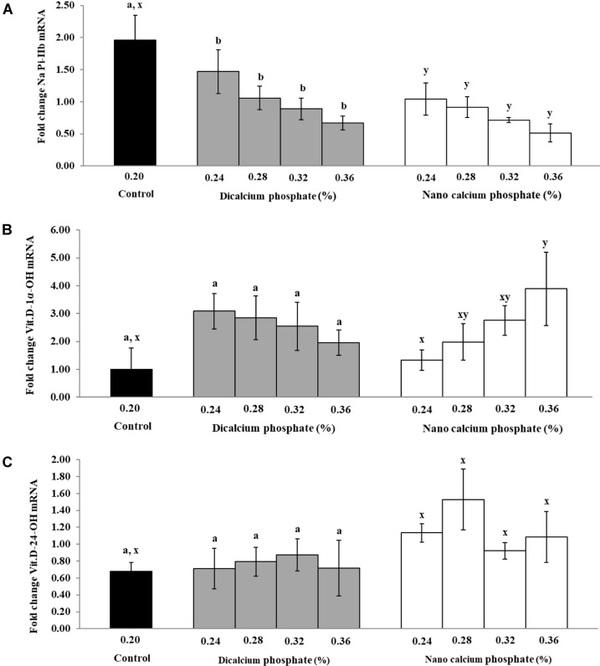
Figure 3. Expression of intestinal NaPi-IIb (A), kidney vitamin D-1α-hydroxylase (B), and kidney vitamin D-24-hydroxylase (C) mRNA in chicks fed various levels of phosphorus in the diet. Chicks were fed diets containing 0.20 (control), 0.24, 0.28, 0.32, and 0.36% available phosphorus from dicalcium phosphate and nanocalcium phosphate sources from 0 to 14 D. The abundance of NaPi-IIb (sodium phosphate cotransporter IIb) mRNA in duodenal mucosa, Vit.D-1α-OH (vitamin D-1α-hydroxylase) mRNA in kidney and Vit.D-24-OH (vitamin D-24-hydroxylase) mRNA in kidney was determined by quantitative PCR. Analysis was performed separately for dicalcium phosphate (control plus 4 dicalcium phosphate levels) and nanocalcium phosphate (control plus 4 nanocalcium phosphate levels). Bars (means ± SE) without common letters (a, b) are different for dicalcium phosphate (P ≤ 0.05) and bars without common letters (x, y) are different for nanocalcium phosphate (P ≤ 0.05).
In the kidney, there was an increase in mRNA abundance for vitamin D-1α-hydroxylase at 0.36% Nano P compared with control (0.20%) (Figure 3B). There was no difference in vitamin D-1α-hydroxylase mRNA abundance between 0.20% P and all other Dical P concentrations. For vitamin D-24-hydroxylase, there was no difference in mRNA abundance between control (0.20%) and Dical P and Nano P levels (Figure 3C). The increase in vitamin D-1α-hydroxylase mRNA in the current study was observed only at the highest level of Nano P and not with Dical P. This difference may be because of differences in solubility due to particle size between Nano P (< 100 nm) and Dical P (500– 1,000 μm). The increase in vitamin D-1α-hydroxylase and no change in vitamin D-24-hydroxylase could potentially lead to an increase in the concentration of active 1,25-dihydroxy vitamin D. When the vitamin D analog, 1α-hydroxy-vitamin D3, was fed to chicks, it was rapidly metabolized to 1,25-dihydroxy vitamin D (Edelstein et al., 1978) and resulted in an increase in growth performance, plasma Pi concentration, and Na Pi-IIb mRNA abundance (Han et al., 2009). Moreover, 25-OH vitamin D3 increased both vitamin D-1α-hydroxylase and NaPiIIb mRNA abundance in broiler chickens from days 1 to 14, thereby promoting active Pi absorption in the duodenum of broilers (Han et al., 2018).
In conclusion, during the starter period, Dical P and Nano P sources significantly increased performance, TAW, and TAP of broiler chicks. In addition, there were no differences between Dical P and Nano P for BW, FI, TAW, and TAP of chicks, indicating that Dical P and Nano P had equivalent bioavailability. In the intestine, mRNA abundance of NaPi-IIb mRNA decreased with increasing dietary Dical P and Nano P; whereas in the kidney, mRNA abundance of vitamin D-1α-hydroxylase was increased by 0.36% Nano P, while vitamin D24-hydroxylase mRNA abundance was unaffected by Dical P and Nano P. Although Dical P and Nano P showed the same bioavailability, they showed a differential effect on vitamin D-1α-hydroxylase gene expression but not intestinal NaPi-IIb gene expression.