Introduction
Avian influenza viruses (AIV) infect animals and humans [1] and thus are of major relevance under the one health context. They are enveloped viruses belonging to Alphainfluenzavirus from Orthomyxoviridae family [2]. These negative sense and single-stranded RNA viruses present a genome that is fragmented into eight segments which encode for eleven proteins. These proteins possess individual function or form complexes during viral replication. The mechanisms underlying AIVs evolution, comprising antigenic shift and drift, point mutations, reassortments and others, are induced mainly by their segmented genome and the receptor specificity, including a wide host range into the animal kingdom [3].
Hemagglutinin (HA) and neuraminidase (NA) are structural proteins responsible for attachment and viral release, respectively. These proteins play a fundamental role in AIV replication. In addition, HA and NA have also been used for viral classification into subtypes. Thus, eighteen HAs and eleven NAs have been described to date [4]. From these, sixteen types of HA (H1-16) and nine of NA (N1-9) were identified in avian species, including wild and migratory birds from Anseriformes and Charadriiformes orders [5]. Other two subtypes, H17N10 and H18N11, have been identified in bats from Guatemala and Peru [6].
Most AIV subtypes have been classified as low pathogenicity avian influenza viruses (LPAIV) and generally induce minimal impact in the health of domestic and wild birds, although there have been reports of sporadic cases in humans related to H5, H6, H7, H9 and H10 avian subtypes [7]. Animal reservoir carrying these viruses spread AIV on the ecosystem, facilitating an effective transmission between vulnerable hosts. Furthermore, genetic changes in relevant proteins, such as HA and NA, can turn them into highly pathogenic avian influenza viruses (HPAIV) with high consequences for other species [8].
Infections by HPAIV have a major social and economic impact in poultry because it can result in massive deaths in the farms, and has a potential for causing disease in humans [9, 10]. In addition, global population expansion has provided new opportunities for spillover events. Altogether, active epidemiological surveillance under a one health approach is essential to develop novel strategies that will allow us to implement measures in a concerted manner before epidemics begins [11]. Hence, it is essential to get a better understanding of viral circulation patterns in our ecosystem, providing fundamental information for AIV risk assessment and detecting probable hotspots of novel AIV emergence [12].
Waterfowl has been considered the main natural reservoir for all AIV subtypes representing an important source for transmission to other species [13]. Moreover, Peru has a central location within the migration route of wild birds in Americas, and therefore is of relevance for viral spreading [14]. Hence, our major objective was the isolation and genetic subtyping and characterization of the AIV circulating in wild birds in Peru, using the HA and NA genetic information, and establishing relationships to other isolates previously detected in America.
Materials and methods
Experimental design and samples
A descriptive, qualitative, cross-sectional study of active epidemiological surveillance for avian influenza in animal reservoir in the Coast of Lima (Peru) was conducted in the period of March 2019 and March 2020. Sampling was carried out targeting different population of waterfowl species belonging to Charadriiformes and Anseriformes orders. This included several residents and migratory waterfowl in Peruvian territory. Fecal samples were collected from the ground using swabs and Universal Transport Media (UTM) (Copan Diagnostics Inc., USA) in cool chain boxes. Samples were taken in 5 natural resting areas for waterfowl in Lima (Puerto Viejo 76.7026578˚W 12.5714006˚S, Pantanos de Villa 76.9949416˚W 12.2065568˚S, Albufera de Medio Mundo 77.6626868˚W 10.9353162˚S, Laguna La Encantada 77.5522327˚W 11.1364147˚S and Poza de La Arenilla 77.1590185˚W 12.0721548˚S). Avian species were determined under close visual observation and photographic record, and classified according to phenotypic characteristics. Thus, fecal samples were taken only when the avian species could be determined.
Samples were transported to the Avian Pathology Laboratory of the School of Veterinary Medicine, Universidad Nacional Mayor de San Marcos (Lima). Fecal samples were processed and clarified by centrifugation (8000 x g for 10min) and supernatants were filtered through 0.22-micron syringe filters. Filtered samples were then pooled according to species, collection date and geographical location into 4 to 5 samples per pool, supplemented with 1000 U/mL Penicillin G, 1 mg/mL Streptomycin and 10 μg/mL amphotericin B, and incubated at 4˚C for 1 hour prior to inoculation into embryonated eggs.
Virus isolation in embryonated chicken eggs
Viral isolation was performed by inoculation of 0.2 mL of pooled samples in ten-day-old specific-pathogen-free (SPF) embryonated eggs through allantoic sac route in five replicates. This process was followed by incubation at 37˚C for 120 hours [15]. Embryo survival was checked daily. Allantoic fluid from each sample was tested by hemagglutination assay as initial screening. Positive samples were titrated using the same technique by serially diluting with two-fold volume of PBS (pH 7.4) and then incubating with equal volume of 1% chicken red blood cells (RBC). After positivity evidence, the positive pool had their samples evaluated individually [16].
Viral detection
Rapid immunochromatographic assay (QuickVue Influenza A+B Test, US) was used for rapid identification of Influenza A virus in allantoic fluid samples that tested positive for hemagglutination test. For confirmation, we used a PCR-based method to amplify the NP gene from the viral genomic material. Thus, the viral RNA was extracted from 140 μL of allantoic fluid using QIAamp Viral RNA Mini Kit (Qiagen, US), according to manufacturer instructions. This was followed by a step of reverse transcription using GoScript™ Reverse Transcription System kit (Promega, USA). In this step, we used the primer described by Hoffman et al. [17] (Uni-12 5’-AGCAAAAGCAGG-3’) that allows amplification of all influenza A segments. NP gene was detected by PCR using GoTaq1 G2 Green Master Mix (Promega, USA) and primers described by Wright et al. (1995) [18] Anp-1 (5’- ATCACTCACTGAGTGACATC-3’) and Anp-2 (5’-CCTCCAGTTTTCTTAGGATC-3’) for 306-bp. The thermal cycling conditions were 94˚C for 3 minutes; 35 cycles of 94˚C for 30s, 58˚C for 30s and 72˚C for 60s; and 72˚C for 5 minutes. PCR products were electrophoresed on 1.5% agarose gels and visualized by ethidium bromide staining.
Next generation sequencing (NGS)
First strand cDNA was prepared using SuperScript™ III First-Strand Synthesis kit (Invitrogen TM), following manufacturer recommendations and using the primer FR20RV (5’- GCCGGAGCTCTGCAGATATC-3’) prior to library preparation [19, 20]. The libraries were prepared using Nextera XT method and sequenced on a MiSeq platform using paired 150 base read chemistry (Cambridge Technologies, Worthington, MN, USA).
Assembly and annotation
The raw data was submitted for end trimming using Trimmomatic, a flexible tool for Illumina NGS data [21] and quality control evaluation based on Phred quality scores [22]. Reads that presented a high Phred score were submitted for de novo genome assembly (DISCOVAR) [23]. Segments that were not properly assembled were submitted to a new round using PRICE [24]. The annotation was performed using “Influenza Virus Resource” tool, from the “National Center for Biotechnology Information”–NCBI, available at https://www.ncbi.nlm.nih.gov/ genomes/FLU/annotation/. After annotation, the segments were edited manually.
Sanger’s sequencing
For some isolates, complete nucleotide sequences of the 5’-end and 3’-end non-coding and coding regions of few gene segments were not obtained by NGS. Therefore, primer pairs were designed to amplify the non-coding and coding sequences of these gene segments by RT-PCR, as described earlier [25, 26]. The resulting amplicons were purified and DNA was directly sequenced or cloned into a pGEM-T vector, and the plasmid DNA was sequenced using Sanger’s dideoxy chain termination method. (Institute of Marine and Environmental Technology, Baltimore, MD, USA).
Thus, complete nucleotide sequences for the whole genome of Peruvians AIVs were attained, namely PE-01 to PE-5, and the data was deposited into the GenBank.
NA and HA subtyping and phylogenetic analysis
Following sequencing, we focused on the subtyping of AIV isolated based on the HA and NA genetic analysis. Each HA and NA segment assembled was submitted to genetic characterization using MEGA [27]. Criteria for databank construction considered the evolutionary pattern of AIV in the ecosystem (host, space and time). The coding region of HA and NA from all AIV subtypes from America available in the webpage of National Center for Biotechnology Information (NCBI), Influenza Virus Genome Set (https://www.ncbi.nlm.nih.gov/genomes/FLU/Database/ nph-select.cgi?go=genomeset) were included and sequences that presented degenerated nucleotide were excluded. The alignment was conducted using MAFFT online service (https://mafft.cbrc.jp/ alignment/server/) according to default parameters and the aligned sequences were posteriorly manually edited using BioEdit 7.2 software (https://bioedit.software.informer.com/7.2/). Phylogenetic analysis was performed using MEGA X (https://academic.oup.com/mbe/article/35/6/1547/ 4990887) determining the best evolution model by using the jModelTest2 software; clustering was obtained using the maximum-likelihood (ML) method under General Time Reversible gamma distribution and proportion of invariable sites parameters and 1,000 replicates of bootstrap.
Results
To survey the presence of AIVs in wild birds from Peru, 421 fecal samples were inoculated in SPF chicken embryos and tested by hemagglutination assay (HA), which was performed by mixing equal volume of allantoic fluid and chicken red blood cells (1:1 dilution). Out of these, eight samples tested positive by HA. Further screening of all eight samples by immuno-chromatography and by RT-PCR amplification of the NP gene evidenced only five positive samples of AIVs (Table 1, S1 Fig).
From five AIV samples collected from the wetlands; two were from Poza de la Arenilla, two from Pantanos de Villa and one from La Encantada, all located in Lima province. Two positive samples were isolated from two franklin gulls in Poza de la Arenilla; one from a kelp gull and one from a whimbrel in Pantanos de Villa; and one from a mallard in La Encantada. A summary of these data, including general information about species and location is presented in Table 2.
Table 1. Status of samples tested positives by hemagglutination test followed by confirmation using rapid antigen and PCR tests for influenza A virus.
Table 2. Twenty-one different avian species identified as possible reservoir of avian influenza virus in Lima Metropolitan Region, Peru, Pacific flyway, South America.
Following detection of these AIV isolates, we performed the whole genome sequencing (WGS), which allowed us to characterize and identify close genetic relatives for each viral segment. Here, we focused on the annotation of HA and NA which reveal that our PE isolates correspond to the following subtypes: H6N8 (PE-01), H2N6 (PE-02), H6N2 (PE-03), H13N6 (PE04) and H6N2 (PE-05) commonly found in wild birds. The information related to accession numbers and the closest relatives for each gene segments is presented in Table 3.
Table 3. Whole genome sequences of AIV isolates obtained in Peru and their closest relatives.
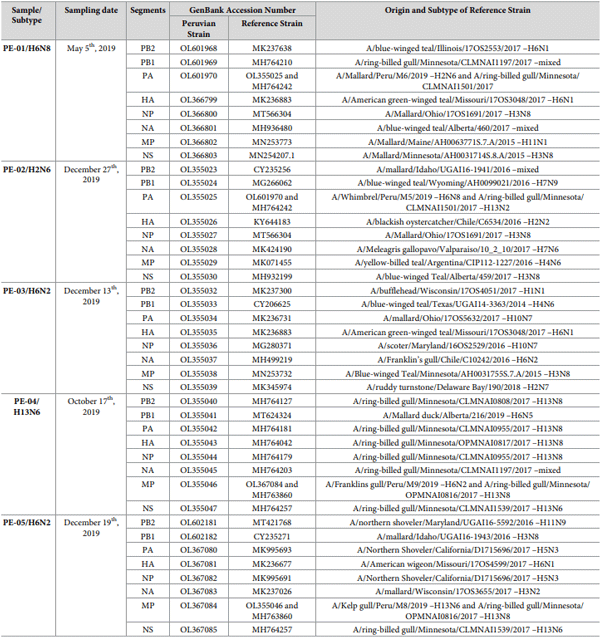
An amino acid analysis was conducted and the list of amino acid substitutions for each HA and NA segments, including additional information is presented in Table 4 and Table 5, respectively. Using HA sequences, we deployed multiple phylogenetic trees, including those HA genetic information from virus subtypes that have been circulating in America. Using Mega 11, we created subtree versions of the complete phylogenetic analysis, which are shown in Figs 1 (H2), 2 (H6) and 3 (H13). Thus, H2 (PE-02) has emerged within a north American clade including isolates from 2011–2015 (Fig 1). All H6 genes (PE-01, PE-03 and PE-05) share a common ancestor and formed a sister group with another monophyletic clade formed by three isolates from US identified in 2015 (ALX30476, H6N1; ALX30488, H6N1 and ALX30464, H6N2) (Fig 2). The H13 (PE-04) sequence was more similar with a mixed strain from USA/2015 and a strain H13N8 identified in the US in 2017 (US/Minnesota/2017) with a 99.47% of amino acid identity (Fig 3). The phylogenetic tree showed a monophyletic group between our isolate with other two isolates from US identified in 2015, a mixed strain APH09815 and a strain H13N8 APY16670. Interestingly, we found multiple amino acid changes compared to their closest relatives. Thus, H2 (PE-02) has multiple changes which had the larger number of changes among our isolates and were also evidenced in the phylogenetic tree since that branch was more dissimilar than others in that group. Other HAs have also shown some amino acid changes but in lower proportions. In contrast, one of our H6N2 isolates (PE-03) did not evidence changes compared to its closest relative.
Table 4. Amino acid substitutions in the Hemagglutinin protein of AIV isolated from wild birds in Peru.
Table 5. Amino acid substitutions in the Neuraminidase protein of AIV isolated from wild birds in Peru.
Fig 1. Phylogenetic tree of HA subtype H2 of an isolate detected in a kelp gull in Peru, and others detected in America. The tree was generated using a maximum likelihood method with 1000 replicates of bootstrap using GTR+G +I as nucleotide substitution model. They were included in the tree with the Peruvian strain (PE-02) and 256 sequences of complete coding region of HA subtype H2 that was detected in America through all time. A subtree of the complete phylogenetic analysis, including the isolate identified in our study is shown. H2 sequences from United states isolates (blue), Canada (green) and Peru (red) are deployed. A collapsed group of North American H2 sequences is shown in black triangle. The complete phylogenetic tree is shown at the side for reference.
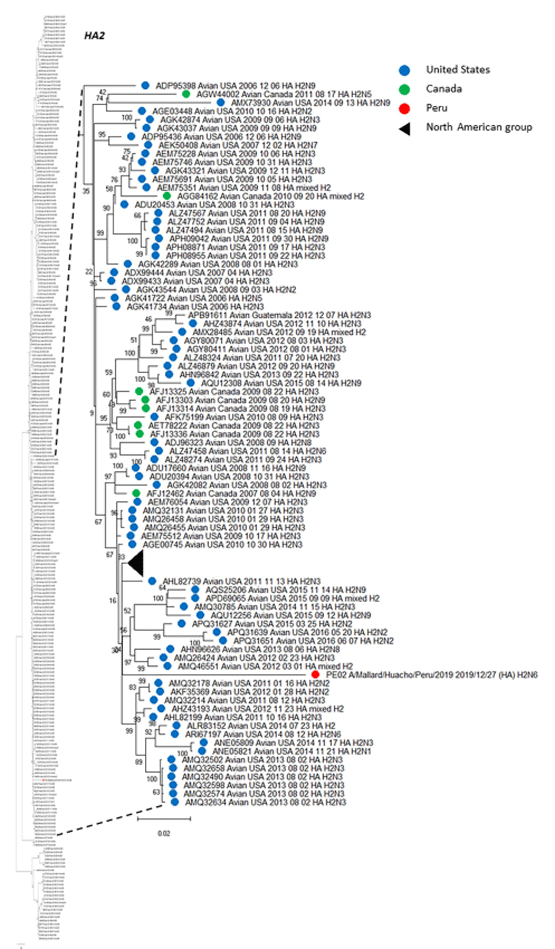
Fig 2. Phylogenetic tree of HA subtype H6 of three isolates detected in whimbrel and franklin gull in Peru, and others detected in America. The tree was generated using a maximum likelihood method with 1000 replicates of bootstrap using GTR+G+I as nucleotide substitution model. They were included in the tree with the Peruvian strains (PE-01, PE-03 and PE-05) and 477 sequences of complete coding region of HA subtype H6 that was detected in America through all time. A subtree of the complete phylogenetic analysis, including those isolates identified in our study is shown. H6 sequences from United states isolates (blue), Argentina (yellow), Brazil (purple) and Peru (red) are deployed. Collapsed groups of North American H6 sequences is shown in black triangle. The complete phylogenetic tree is shown at the side for reference
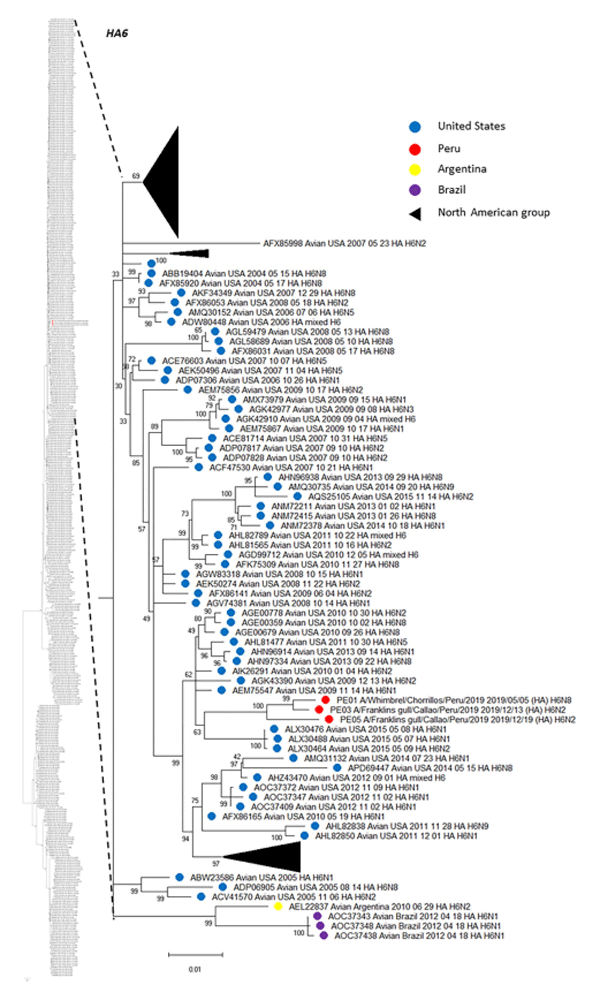
Fig 3. Phylogenetic tree of HA subtype H13 of an isolate detected in a kelp gull in Peru, and others detected in America. The tree was generated using a maximum likelihood method with 1000 replicates of bootstrap using GTR+G +I as nucleotide substitution model. They were included in the tree with the Peruvian strain (PE-04) and 64 sequences of complete coding region of HA subtype H13 that was detected in America through all time. H13 sequences from United States (blue), Canada (green), Argentina (yellow), Chile (orange) and Peru (red) are deployed.
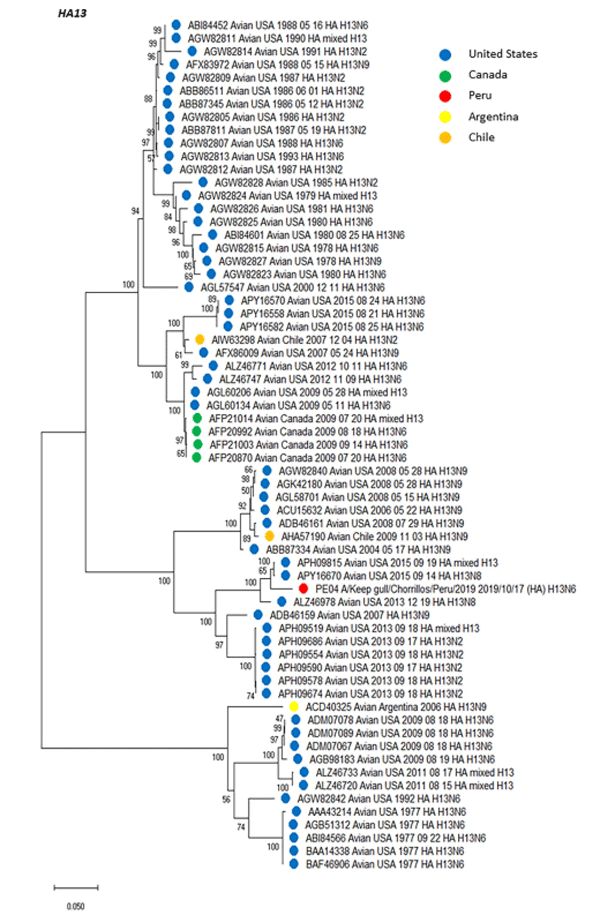
Based on NA sequence analysis, we also deployed phylogenetic trees including that NA genetic information from virus subtypes that have been circulating in America. Similar to HA analysis, Figs 4 (N2), 5 (N6) and 6 (N8) show the phylogenetic relationship among NA genes reported to date, presented as subtree versions of complete phylogenetic trees. Hence, we identified that both N2 from our two H6N2 isolates arose from two divergent ancestors. N2 (PE-05) presented the highest similarity with a strain H5N2 (US/Arkansas/2015), with 99.56% of amino acid identity, while N2 (PE-03), clustered with three different strains from Chile in 2016, presenting 98.69% amino acid identity with the closest relative. Furthermore, the phylogenetic tree showed that the both N2, isolated in the same site from Peru (77.1595302˚W, 12.0717358˚S), from the same wild bird species (Franklin’s gull), sampled one week apart (13th and 19th of December, 2019), grouped in two different clades. For N2 (PE-05), it grouped with all US isolates. The other strain (PE-03) exhibited the closest relationship with those identified in Chile and Argentina (Fig 4). In terms of N6, the one from PE-02 had the highest similarity with a strain from Chile in 2017 (Chile/2017, H7N6), whereas, the N6 sequence from our H13N6 isolate was closely related to the US isolate (US/Minnesota/2017/H13N6) with 99.57% amino acid identity. The phylogenetic tree presented the topology with the same characteristics (Fig 5), showing that Peruvian H13 strain formed a monophyletic group with Chilean strains identified in 2013 (H3N6, ANH21898 and H7N6, ANH21875) and the other, with strains from US also identified in 2013 (four H13N6 isolates, AIN76265, APY16561, ALZ46774, ALZ46750 and a mixed N6, APH09819). Moreover, N8 from PE-04 had more similarity with the US isolate (US/ New Jersey/2017/H5N8) having 99.57% amino acid identity (Fig 6).
Fig 4. Phylogenetic tree of NA subtype N2 of isolates detected in two franklins gulls in Peru, and others detected in America. The tree was generated using a maximum likelihood method with 1000 replicates of bootstrap using GTR +G+I as nucleotide substitution model. They were included in the tree with the Peruvian strains (PE-03 and PE-05) and 1020 sequences of complete coding region of NA subtype N2 that was detected in America through all time. A subtree of the complete phylogenetic analysis, including isolates identified in our study is shown. N2 sequences from United States (blue), Argentina (yellow), Chile (orange) and Peru (red) are deployed. Collapsed groups of North American N2 sequences are shown in black triangles. The complete phylogenetic tree is shown at the side for reference
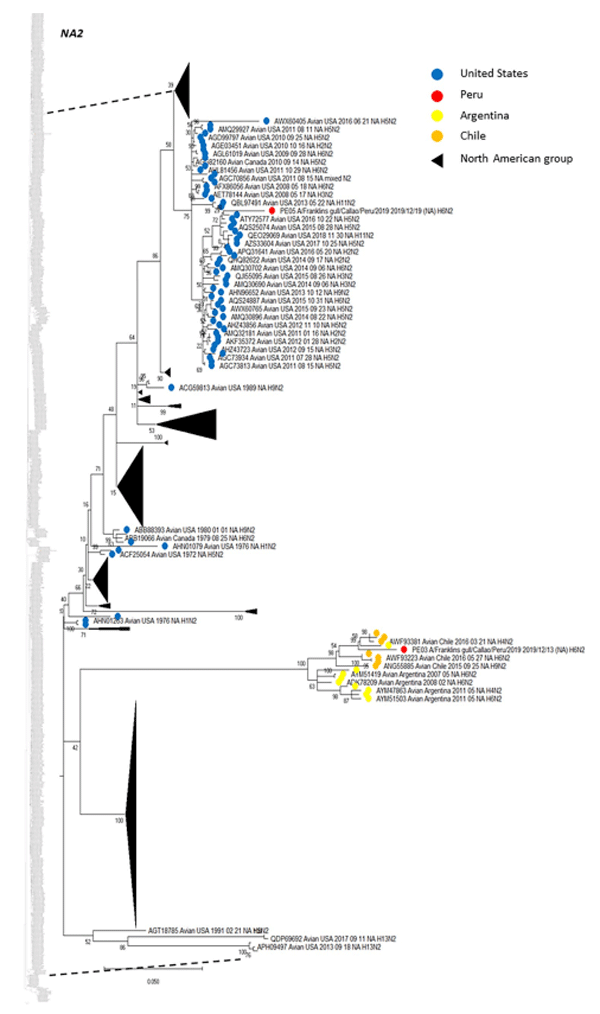
Fig 5. Phylogenetic tree of NA subtype N6 of two isolates detected in a kelp gull and a mallard in Peru, and others detected in America. The tree was generated using a maximum likelihood method with 1000 replicates of bootstrap using GTR+G+I as nucleotide substitution model. They were included in the tree with the Peruvian strains (PE-02 and PE-04) and 585 sequences of complete coding region of NA subtype N6 that was detected in America through all time. A subtree of the complete phylogenetic analysis, including isolates identified in our study is shown. N6 sequences from United States (blue), Canada (green), Chile (orange) and Peru (red) are deployed. Collapsed groups of North American N6 sequences are shown in black triangles. The complete phylogenetic tree is shown at the side for reference
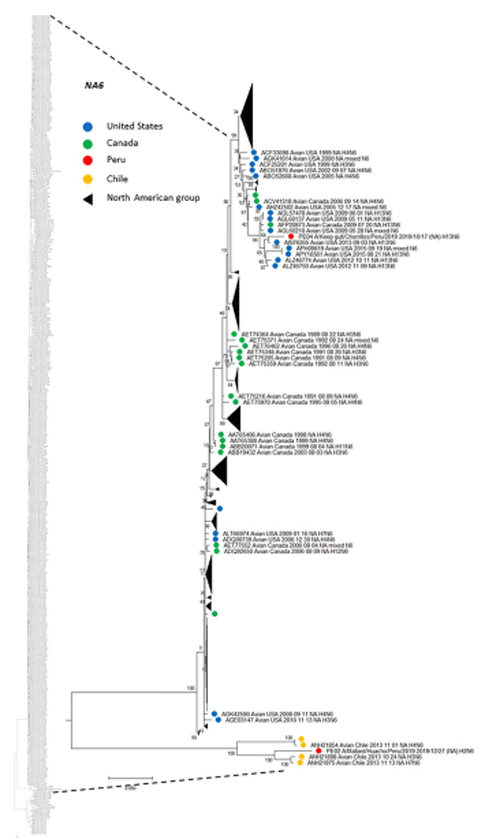
Fig 6. Phylogenetic tree of NA subtype N8 of an isolate from a whimbrel in Peru and others detected in America. The tree was generated using a maximum likelihood method with 1000 replicates of bootstrap using GTR+G+I as nucleotide substitution model. They were included in the tree with the Peruvian strain (PE-01) and 741 sequences of complete coding region of NA subtype N8 that was detected in America through all time. A subtree of the complete phylogenetic analysis, including the isolate identified in our study is shown. N8 sequences from United States (blue), Canada (green) and Peru (red) are deployed. The complete phylogenetic tree is shown at the side for reference
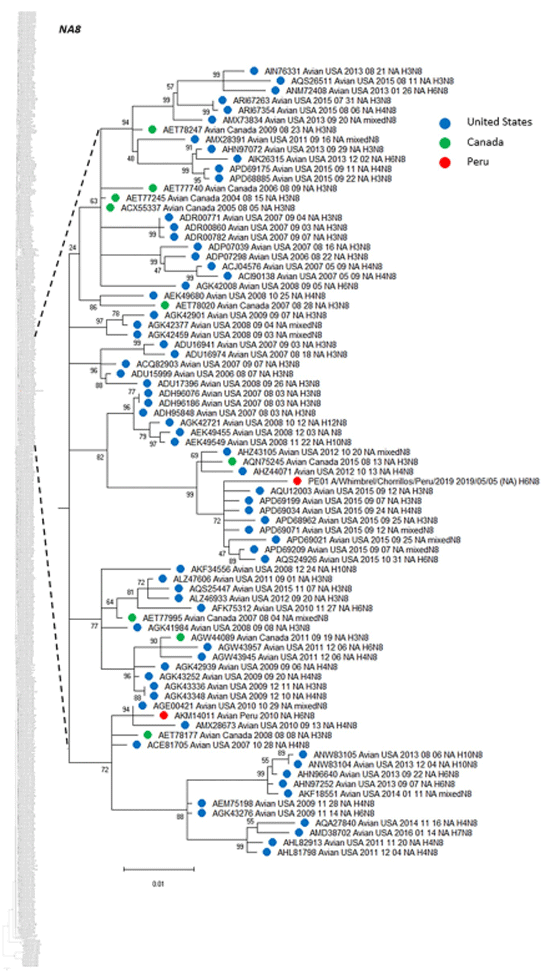
Fig 7. Schematic representation of genetic flow of avian influenza virus isolates obtained in the study. Most of the viral genes has closely related relatives from isolates in North America, reflecting the genetic flow of AIV isolated in Peru comes from relatives in North American countries. Isolates PE-02 (H2N6) and PE-03 (H6N2) has both NA genes with common relatives from South America. In addition, sample PE-02 has also HA and MP from common ancestor from Chile and Argentina, respectively. PE-05 has a MP gene closely related to an isolate from Peru. The Pacific (sky blue), Mississippi (orange) and the Atlantic (green) flyways are shown
Discussion
To conduct surveillance of avian influenza A virus (AIV) circulating among the wild birds in Peru, we collected and tested fecal samples from several species of birds along the coast of Peru. We isolated five AIVs and their whole genome was sequenced. Genetic analysis of HA and NA genes revealed that these viruses are of subtypes H6N8, H13N6, H6N2 and H2N6. Our results also demonstrated a high HA and NA variability in our isolates, as compared to others previously identified, indicating the active viral circulation within the populations assessed. Despite the multiple orders evaluated, Anseriformes and Charadriiformes orders are the main natural reservoir of AIV in Peru. According to Rejmanek et al. (2015) [28, 29], H6N2 and H6N8 have been frequently isolated from mallard and goose (Anseriformes), while H13 and H16 have been exclusively isolated from gulls (Charadriiformes). Our findings show that this pattern varies, which might explain in part, the higher variability of HA and NA observed. Further studies are required to assess the implications of host variation on viral evolution. Nevertheless, all isolates found in the study belonged to subtypes commonly known as low pathogenic avian influenza viruses (LPAIV).
The HA protein present 10−4 amino acid substitutions / site / year under action of purifying selection, mainly driven by the host immune responses [30]. This suggests that viral genetic structure tends to be determined by “space”, “territoriality” under a sympatric speciation model, indicating that evolutionary events within the same migration route are more likely to occur [31]. In our study, sites where we detected those AIV isolates are common geographical sites for migratory birds feeding and resting within their migratory route, considering that these places create potential sites for emergence of novel strains. On the other hand, according to HA specificity in the host cell receptor, each AIV subtype has a group of susceptible animals to infections, and in multiple cases, include humans in the center of this circulation pattern [1]. In this context, public policy actions must consider a one-health dynamics, in which all factors must be considered, from factors such as environmental preservation, animal and human health, since only this perspective will have the possibility of success in regional and global epidemiological surveillance for emergency containment of highly pathogenic strains.
Peru is a biodiverse country for multiple avian species and a special location to identify new strains of AIV since it is located right at the crossroads of Pacific and Mississippi migratory routes. Our study identified five different isolates, including four subtypes obtained from four different migratory bird species. Our isolates presented hemagglutinin whose subtypes originated from US (H2, H6 and H13 presented closest relationship with isolates identified in US and Canada); while the neuraminidase of our isolates originated from Chile (N2), Chile and US (N6). Since our isolates were obtained from migratory birds, these findings suggest that Peru, with its geographic position is an important site for emergence of novel reassortants of AIV. A schematic representation of the genetic flow across Americas involved in the evolution of AIV in Peru is shown in Fig 7. The H2 identified in a mallard presented a special attention since it could be transmitted to humans directly or through domestic poultry infections and possibly associated to cause the next flu pandemic [32, 33]. However, little is known in terms of viral interaction with its natural host. AIV H2 produced a severe pandemic in 1957 and was reported circulating in North America in the end of 90’s [34]. On the other hand, the H6 was identified in two different species, in a whimbrel and in a Franklins’ gull. Interestingly, domestic poultry has been reported to be extremely susceptible to this subtype [35], representing an economic and social threat. H6 segment has been intensely identified in mallard from Canada and presents global distribution. Moreover, H6N2 and H6N8 subtypes have been reported in several parts of America for more than forty years. For instance, H6N2 was identified in USA (1986–2015), in Mexico (2007) and Argentina (2010) and Chile (2016–2017) [36]. On the other hand, H6N8 has been circulating in America between 1977–2016 in USA, detected in Argentina and Peru in 2021; and in Chile, in 2016 and 2017. Thus, H6N8 is described as a high-priority subtype [29]. Similar to H2 subtype, H6 has been used experimentally in ferrets to produce vaccine candidates [37]. Finally, the H13 identified in a kelp gull can be spread to domestic poultry and other mammals. Interestingly, H2 and H13 have shown global distribution, while H2 subtype has a closest phylogenetic relationship with H5, indicating a more recent differentiation between these subtypes. This close phylogenetic relationship between H2 and H5 might induce events for viral reassortments.
In regard to NA, N2 subtype isolated from two Franklin’s gulls has shown to infect swine, marine mammals, dogs, poultry, and humans. For instance, H9N2 subtype has presented high prevalence in domestic and wild birds and has been isolated from swine and humans, which has strong ability for inter-species transmission [38]. N6 has also been described as a high-priority subtype [29] and was identified in two different strains in our study, one obtained from an Anseriformes (mallard) and other from a Charadriiformes (kelp gull). This N6 subtype needs special attention because in addition to infecting aquatic mammals, it can infect swine and thus suitable for genetic changes [39]. Finally, N8 subtype has been widely identified in America, sometimes in association with the subtype H6, as we found in our study, and is considered as a high-priority subtype [29].
Some evidence highlights that strains identified in the same flyway are more similar between them than those found in others flyway during a period [32]. The America continent presents three different flyways: the Pacific, the Mississippi and the Atlantic. In our study, samples were obtained from animals from the Pacific flyway. Further studies are required to assess the viral circulation in flyways crossing other areas in South America. Hence, considering the spatial evolution, AIV strains seem to be spatially structured. Nevertheless, in multiples places, the overlapping of the flyways facilitates the genic flow and the possibility to generate novel strains. Additionally, in the northern region such as Canada and Alaska, there is a confluence of multiple flyways from several parts around the world and thus AIV strains do not show spatial genetic structure [40]. Finally, our results indicate that multiple AIV subtypes are circulating in wild birds in Peru. Even though those LPAIV subtypes have been previously detected, this work has revealed that these isolates possess unique features at the nucleotide and protein level. Although we have not defined what the implications of these amino acid changes represent on HA and NA function, our results provide novel insights into AIV evolution for genetic surveillance.
Conclusion
Our study shows the active circulation of multiple AIV subtypes in wild birds in Peru. Despite their close genetic relationship with other common subtypes previously found in America, our findings have identified that these isolates have gained multiple unique changes during the viral evolution in America. Altogether, this work has shed the light on the importance of Peru, which confers ecological advantages as relevant geographic location for the emergence of novel AIV in America with pandemic risk.
This article was originally published in PLoS ONE 17(6): e0268957. https:// doi.org/10.1371/journal.pone.0268957. This is an Open Access article distributed under the terms of the Creative Commons Attribution License.