1Harrisvaccines Inc., Ames, IA 50010, USA
2Immunobiology Program, Iowa State University, Ames, IA 50011, USA
3Department of Veterinary Microbiology and Preventive Medicine, College of Veterinary Medicine, Iowa State University, Ames, IA 50011, USA
4Department of Animal Science, College of Agriculture, Iowa State University, Ames, IA 50011, USA and
5 Department of Veterinary Diagnostic and Production Animal Medicine, College of Veterinary Medicine, Ames, IA, 50011, USA
© Cambridge University Press 2012
ISSN 1466-2523- doi:10.1017/S1466252312000011
Received 23 November 2011; Accepted 26 January 2012
Abstract
The alphavirus replicon technology has been utilized for many years to develop vaccines for both veterinary and human applications. Many developments have been made to the replicon platform recently, resulting in improved safety and efficacy of replicon particle (RP) vaccines. This review provides a broad overview of the replicon technology and safety features of the system and discusses the current literature on RP and replicon-based vaccines.
Keywords: alphavirus biology, dendritic cell tropism, safety, DIVA
Introduction
Traditionally, veterinary vaccines consisted of either modified live or inactivated preparations. Modified live vaccines (MLV) have the possibility of reverting to virulence with subsequent spread among surrounding animals (Botner et al., 1997; Madsen et al., 1998). Inactivated vaccines often generate insufficient cell-mediated immunity required for protection and so must be combined with adjuvants that are able to induce the required immune response (Minke et al., 2004; Meeusen et al., 2007). Thus, there has been a focus on 'second-generation' vaccines, some of which have already been licensed for commercial veterinary use (Meeusen et al., 2007).
Alphavirus replicon-based vaccines represent a viable option for next-generation vaccine development. To date, alphavirus replicon-based vaccines have not been approved by any government regulatory agency for use in animals or humans. Alphavirus replicon particles (RPs) are single-cycle, propagation-defective particles that are not able to spread beyond the initial infected cells. RPs are produced by removing the alphavirus structural genes from the replicon RNA vector and replacing them with a heterologous gene or genes of interest. Helper genes can be provided in trans along with replicon RNA and cotransfected into permissible cells, resulting in the packaging of the replicon RNA. RP vaccines have been evaluated in many different species of animals as well as humans with a proven record of safety and efficacy. These vaccines are capable of inducing robust and balanced immune responses and offer many other advantages that ideal vaccines possess.
Alphavirus biology
The Alphavirus genus belongs to the Togaviridae family and contains 28 virus species (Griffin, 2007). Alphaviruses are positive-sense, single-stranded RNA viruses with a genome of approximately 11.5 kb in length. The positivesense genome contains two open reading frames (ORFs) and encodes four non-structural proteins and five structural proteins (Strauss and Strauss, 1994). The 50 ORF encodes four non-structural proteins (nsp1–4) and the 30 ORF encodes the virus structural proteins (capsid and glycoproteins (E3, E2, 6 K and E1)) (Strauss and Strauss, 1994; Rayner et al., 2002). The non-structural proteins are translated from the positive-sense genomic RNA and function to transcribe full-length negative-sense RNA (Fig. 1). Translation of the nsp1–3 polyprotein is terminated by an opal stop codon located between nsp3 and nsp4; the polyprotein nsp1–4 is produced when translational read through occurs at the nsp3–4 junction (Kinney et al., 1988), although there are notable exceptions where the opal stop codon is replaced by a sense codon, as is the case for strains of Semliki Forest virus (SFV), O'nyong-nyong virus (ONNV) and Sindbis virus (SIN) (Takkinen, 1986; Levinson et al., 1990; Strauss and Strauss, 1994).
Fig. 1. Alphavirus genome organization and replication strategy.
Fig. 2. Alphavirus RP vaccine and packaging system. Portions of this image courtesy of AlphVax Inc. (Research Triangle Park, NC, USA).
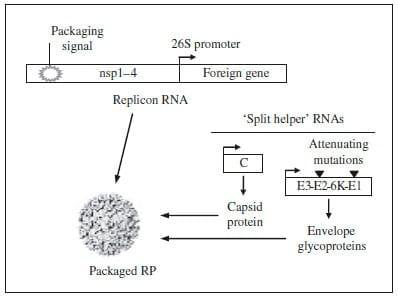
The negative-sense RNA is a template for both additional genomic RNA as well as 26S subgenomic mRNA. The 26S promoter is located between the two ORFs on the negative-sense RNA and is recognized by the non-structural proteins for transcription of a sub-genomic mRNA, from which the structural proteins are translated. This 26S mRNA is produced in 10-fold molar excess when compared to genomic RNA (Strauss and Strauss, 1994). The structural proteins are translated from the subgenomic 26S mRNA as a polyprotein that is subsequently co-translationally and post-translationally cleaved to release the capsid protein and the two mature envelope glycoproteins (E1 and E2) (Jose et al., 2009). Foreign genes of interest can be inserted in the place of alphavirus structural genes in cDNA clones, generating a selfreplicating RNA (replicon) capable of expressing the foreign gene when introduced into cells. The selfamplifying replicon RNA directs the translation of large amounts of heterologous protein in transfected cells, reaching levels as high as 15–20% of total cell protein (Pushko et al., 1997). The replicon RNA can be packaged into RP by supplying the structural genes in trans in the form of capsid and glycoprotein helper RNAs (Fig. 2) (Pushko et al., 1997; Kamrud et al., 2010b). When the helper and replicon RNAs are co-transfected into permissible cells, the replicon RNA is efficiently packaged into single-cycle, propagation-defective RP that are morphologically indistinguishable from native alphaviruses (our unpublished observation). Importantly, only the replicon RNA is packaged into RP, as the helper RNAs lack the packaging sequence required for encapsidation (Volkova et al., 2006). Therefore, the resulting RP are propagationdefective and are incapable of producing progeny particles or virus.
Several features of alphavirus RP make them attractive for vaccine development: (1) a proven record of safety; (2) high expression levels of heterologous genes; (3) dendritic cell (DC) tropism; (4) protective and balanced immune responses; (5) multivalent vaccine construction; (6) resistance to anti-vector immunity; (7) commercial vaccine production; and (8) differentiating infected from vaccinated animals (DIVA).
Safety
Replicon vectors have been developed from several different alphaviruses, including Venezuelan equine encephalitis virus (VEEV), SIN and SFV (Liljestrom and Garoff, 1991; Bredenbeek et al., 1993; Pushko et al., 1997). Replicon vectors lack the alphavirus structural protein genes but retain the non-structural genes and the 26S sub-genomic promoter. Early production of RP was hampered by recombination events that resulted in the generation of replication-competent virus (RCV) (Weiss and Schlesinger, 1991; Bredenbeek et al., 1993; Rayner et al., 2002); first-generation helper RNAs encoded the capsid and glycoprotein genes on the same RNA molecule, and thus only required one recombination event to create RCV (Geigenmuller-Gnirke et al., 1991; Berglund et al., 1993; Pushko et al., 1997). The probability of this event occurring was greatly reduced by separating the helper RNAs onto two separate RNAs ('split helper' system). Pushko et al. were able to demonstrate increased safety of the system by decreasing recombination events leading to the production of infectious virus when utilizing the split helper system (Pushko et al., 1997). When both helper genes (capsid and glycoprotein) were present on a single RNA and co-electroporated into cells with replicon RNA expressing a heterologous gene, infectious virus was typically recovered, even to levels as high as 2x105 PFU/ml (Pushko et al., 1997). However, when the helper RNAs were split into two separate RNA molecules, there was no recovery of infectious virus by plaque assay, blind passaging, or intracerebral inoculation of mice (Pushko et al., 1997). Similar split helper systems have also been developed for SIN and SFV helper RNAs, resulting in no recovery of infectious virus (Frolov et al., 1997; Smerdou and Liljestrom, 1999).
The split helper system greatly reduces the occurrence of RCV, as separation of the helper RNAs requires two independent recombination events to occur for generation of RCV (Pushko et al., 1997; Smerdou and Liljestrom, 1999). Initially, helper RNAs were designed to contain a 26S promoter downstream of the 50-untranslated region because of the significant impact the promoter has on the production of high levels of subgenomic transcripts. However, a recent study demonstrated that the 26S promoter is not required for functional helper RNAs (Kamrud et al., 2010a). Removal of the 26S promoter results in helper RNAs that are not independent transcriptional units, and further reduces the probability of functional recombinations between the replicon and helper RNAs. In the same study, a stop codon was introduced at the 30-end of the capsid gene in place of the chymotrypsin-like cleavage site (Kamrud et al., 2010a, b). This mutation negates the cleavage activity of the capsid protein, adding another safeguard against functional recombination. Thus, helper RNAs lacking 26S promoters that have been manipulated to include a capsid stop codon have a reduced probability of functional recombination compared with the standard split helper RNA system (Kamrud et al., 2010a). The introduction of the split helper system and subsequent modifications were significant advances in replicon technology that have facilitated RP vaccine evaluation in vivo without risk of reversion to virulence.
Human preclinical evaluations of cytomegalovirus (CMV) and influenza RP vaccines have been completed with no adverse effects reported in toxicology studies. An HA/NA influenza RP vaccine was administered to white rabbits four times with no toxic side effects and no relevant clinical parameter differences observed between RP and placebo vaccinated animals (Hubby et al., 2007). This CMV vaccine has recently been evaluated in a Phase I human study where the vaccine was well tolerated with only mild to moderate local reactogenicity and minimal systemic reactogenicity even after three doses (Bernstein et al., 2010).
Other studies have used the mouse model of intracranial (IC) injection to demonstrate the safety of RP. IC injection of VEEV RP resulted in only transient pathology (1–2 days) and weight loss (1 day) with a rapid return to pre-injection status (Kowalski et al., 2007). The neurovirulence of both SIN and SFV RP expressing LacZ has also been evaluated following IC inoculation. Beta-galactosidase activity was detectable in brains for 14–28 days, but no morbidity, neuropathology, or loss of motor skills was observed in either study, indicating a lack of reversion to the parental virus strains (Altman- Hamamdzic et al., 1997; Lundstrom et al., 1999). A biodistribution study done with the same SFV RP system demonstrated that, following IC injection, there was no evidence of RP RNA co-localization to either the liver or the brain after 15 days, and no abnormalities were observed during histopathological examination (Morris- Downes et al., 2001). These results correlate with safety studies we have completed in pigs and mice following injection with a VEEV RP vaccine expressing an H3 influenza gene, indicating a lack of shedding and spread of RP RNA and a lack of reversion to virulence following vaccination (Vander Veen et al., 2012).
The current molecular features of the RP system result in safe vaccines. However, if in such a rare circumstance the perfect pair of recombination events did occur in the exact order and positions needed (Kamrud et al., 2010a), the resulting RCV would theoretically be no more virulent than the parent strain being used as the vaccine vector. The attenuated VEEV strain TC-83 (commonly used in replicon vector development and production) has been used extensively to vaccinate military personnel and lab workers against VEEV infection (Alevizatos et al., 1967; Burke and Ramsburg, 1977). A retrospective study of hundreds of humans who received the vaccine from 1976 to 1990 indicated that TC-83 vaccine caused some transient reactions, but no serious sequelae were reported (Pittman et al., 1996). This strongly suggests that if multiple improbable functional recombination events were to occur during RP production, the resulting recombinant virus would be no more virulent than the infectious parent strain and thus would not have serious public health consequences.
High expression levels of heterologous genes
Pushko et al. demonstrated that transfection of several cell lines with RP expressing the Lassa virus N protein resulted in expression levels of nearly 20% of total cell protein (Pushko et al., 1997). Kamrud et al. engineered the VEEV replicon to allow further optimization of protein yield and replicon packaging efficiency (Kamrud et al., 2007). Internal ribosome entry site (IRES) elements were inserted into the replicon vector downstream of the 26S promoter to allow for cap-independent translation of heterologous genes. In addition, random nucleotide sequences of varying lengths were inserted between the 26S promoter and the IRES element. When compared to null replicons (no IRES) the spacer-IRES replicons expressed protein in some instances at > 50-fold increases (Kamrud et al., 2007). Thus, by varying the length of the spacer sequences used in conjunction with IRES elements, replicons expressing the highest levels of heterologous protein or resulting in the highest RP yield can be readily identified for further vaccine evaluation.
DC tropism
A robust immune response is dependent on accurate and rapid presentation of the antigen to immune effector cells. DC are considered professional antigen presenting cells, thus vaccines that target these cells should induce robust and balanced immune responses. Inoculation of mice with VEEV RP revealed Langerhans cells (DC located in the skin) as the initial cell set to be infected (MacDonald and Johnston, 2000). In humans, VEEV RP have a selective myeloid DC tropism and these DC retain the capacity to acquire the mature phenotype upon migration to the local draining lymph node (Nishimoto et al., 2007). SFV has also been shown to infect Langerhans cells and subsequently migrate to the local lymph node (Johnston et al., 2000). A single amino acid substitution in the E2 glycoprotein of the SIN replicon vector significantly increased the affinity of the particle for human DC, resulting in an increase of major histocompatibility complex (MHC) class II molecules, CD86, and IFN-g secreting cells (Gardner et al., 2000). This natural or enhanced DC tropism of alphavirus RP results in a balanced and protective immune response following RP vaccination.
Protective immunity
The alphavirus replicon system has been used to develop efficacious RP vaccines for both human and veterinary applications. Influenza RP vaccines have been evaluated in chickens, pigs and humans. Complete protection against lethal H5N1 avian influenza challenge was demonstrated in 2-week-old chickens that received a single dose of RP vaccine expressing the homologous HA gene (Schultz-Cherry et al., 2000). Recent reports also demonstrate that protective HI responses are elicited in young pigs following HA RP vaccination (Erdman et al., 2010; Bosworth et al., 2010; Vander Veen et al., 2012). Following homologous challenge, there was a significant decrease in nasal shedding, viral load, rectal body temperatures and pulmonary pathology in HA RP vaccinated animals compared to placebo controls (Bosworth et al., 2010; Vander Veen et al., 2012).
The alphavirus replicon system was also used to rapidly produce a recombinant HA protein vaccine in response to the pandemic H1N1 influenza outbreak in 2009 with similar protection observed following homologous challenge (Vander Veen et al., 2009). Other influenza gene candidates have also been evaluated in the RP system including neuraminidase (NA) and nucleoprotein (NP), with varying results (Sylte et al., 2007; Hubby et al., 2007; Bosworth et al., 2010). Preclinical evaluation of HA and NA RP vaccines have been completed for human trials with no adverse effects observed following toxicology and safety testing, and robust humoral and cellular responses were elicited in mice, rabbits and rhesus macaques (Hubby et al., 2007). In addition to evaluation as influenza vaccine candidates, RP not expressing any heterologous genes (null RP) were able to act as adjuvants and enhance the immunogenicity and efficacy of a trivalent inactivated influenza vaccine in rhesus macaques (Carrol et al., 2011).
In addition to influenza, RP vaccines have been evaluated against several other animal diseases of veterinary importance. Balasuriya et al. used VEEV RP expressing the GL and M proteins of equine arteritis virus (EAV) and demonstrated that these two major envelope proteins are necessary as a heterodimer for the induction of EAV neutralizing antibodies in mice (Balasuriya et al., 2000). Further, horses vaccinated with the GL/M RP vaccine were protected against virulent challenge, while horses receiving RP expressing only the GL or M monomers were not protected from EAV (Balasuriya et al., 2002). Similar research has been completed evaluating a related Arterivirus, porcine reproductive and respiratory syndrome virus (PRRSV), where the GP5/M heterodimer has also been shown to be important for induction of neutralizing antibody (Jiang et al., 2006a, b, c). RP vaccines expressing the PRRSV GP5/M heterodimer have been evaluated in swine with a reduction in viremia observed post-challenge (Mogler, 2009; Mogler et al., 2010). A recent study evaluated RP vaccines that encoded either the glycoproteins of Hendra virus or the glycoproteins of Nipah viruses (Defang et al., 2011), agents of diseases of both veterinary and public health importance. These vaccines were able to induce cross-reactive neutralizing antibodies to both viruses, suggesting that a single vaccine against both viruses may be possible (Defang et al., 2011).
In addition to alphavirus-based RP vaccines, alphavirus vectors can be designed to launch a self-replicating replicon RNA from a DNA plasmid in vivo. Replicon DNA vaccines have been developed for SIN, SFV and VEEV (Dubensky Jr et al., 1996; Kohno et al., 1998; Berglund et al., 1998; Ljungberg et al., 2007). The first step of expression involves RNA polymerase II-initiated transcription of replicon RNA from plasmid DNA in the transfected cells. Currently, the CMV immediate–early promoter is most frequently used (Ljungberg et al., 2007). The second step of expression occurs when the replicon RNA enters the cytoplasm and the heterologous gene of interest is amplified from the native alphavirus 26S subgenomic RNA promoter. Previous SFV- and SIN-based replicon DNA vaccines have been shown to be immunogenic in small animal models (Berglund et al., 1998; Hariharan et al., 1998). A VEEV replicon-based DNA plasmid consistently expressed 3- to 15-fold more protein in vitro and increased humoral responses by several orders of magnitude over a conventional DNA vaccine (Ljungberg et al., 2007). As the transcribed replicon RNA is self-amplifying, increases in cellular and humoral responses were also observed when 100-fold lower doses of replicon DNA were used compared to conventional DNA. Additionally, when the replicon DNA vaccine was used as a prime and VEEV RP expressing the same heterologous gene was given as the boost dose, both humoral and cellular immunity were increased significantly compared to VEEV DNA alone (Ljungberg et al., 2007).
Similar replicon-based DNA vaccines have recently been evaluated in mice and pigs. An SFV replicon-based DNA vaccine has been produced, expressing the E2 glycoprotein of classical swine fever virus (CSFV). Pigs vaccinated with this DNA vaccine elicited low levels of neutralizing antibodies 1 week post-booster vaccination but exhibited decreased clinical symptoms and reduced viremia following homologous challenge when compared to control pigs (Li et al., 2007). When evaluated in a mouse model, this vaccine elicited a specific lymphoproliferative response and an increase in IFN-g and IL-4 CSFV-specific secretion (Zhao et al., 2009a). When the CSFV DNA vaccine was used in a prime/boost regimen with a recombinant adenovirus expressing the homologous E2 glycoprotein, a significant increase in prechallenge neutralizing antibody titers was observed with subsequent protection against heterologous CSFV challenge (Zhao et al., 2009b). An SFV replicon-based DNA vaccine expressing the 1BCD gene of swine vesicular disease virus has also been shown to elicit neutralizing antibodies and lymphocyte proliferation in both guinea pigs and swine (Sun et al., 2007). A SIN virus repliconbased DNA vaccine encoding the rabies virus glycoprotein G induced higher levels of humoral and cell-mediated immunity in mice than the conventional rabies DNA vaccine and comparable to the inactivated commercial rabies vaccine (Saxena et al., 2008). All of the replicon DNA and inactivated virus vaccinated mice were completely protected against lethal challenge while some mice receiving the conventional DNA vaccine did not survive (Saxena et al., 2008). These results indicate that the alphavirus replicon technology is flexible and that replicon RNA can be delivered to the host by several different methods. In addition, replicon technology can be used in conjunction with other recombinant systems to produce more efficacious vaccine regimens.
Multivalent vaccines
One of the advantages of the alphavirus replicon system is that the vector can be genetically modified to express several different genes from either the same or different pathogens. This can be accomplished via several methods, but the most common method is insertion of additional 26S promoter sites downstream of the nonstructural genes. However, not all proteins are expressed at equimolar levels in this design and production levels can depend on the number of genes or gene position in the replicon, so each replicon must be empirically evaluated to determine optimum protein expression (Reap et al., 2007a). Mice that received RP vaccines expressing different combinations of pp65, IE1 and gB proteins under control of three different 26S promoters developed high titers of neutralizing antibody and antigen-specific T-cell responses against CMV (Reap et al., 2007b). Balasuriya et al. produced an RP vaccine co-expressing the GL/M heterodimer that was required for protection against EAV (Balasuriya et al., 2002).
RP vaccines expressing genes from two different pathogens are also able to induce protection against subsequent challenge. An RP vaccine co-expressing the glycoprotein genes of both Ebola and Lassa viruses protected guinea pigs from challenge with both viruses (Pushko et al., 2001). The results obtained were the same as those achieved with RP vaccines expressing only one of the viral glycoproteins, indicating that protective immune responses can be induced against multiple and individual vaccine antigens at similar levels. In other studies, the authors have simply mixed different monovalent RP vaccines together prior to injection rather than produce the vaccine utilizing the multivalent approach of additional 26S promoters (Lee et al., 2006; Hooper et al., 2009). Similar to the previous results, a specific immune response was elicited in mice to each individual RP antigen including Marburg virus, anthrax and botulinum neurotoxin and protection was demonstrated following challenge with Bacillus anthracis and botulinum neurotoxins A and C (Lee et al., 2006). The level of protection against B. anthracis induced by this multivalent vaccine formulation was similar to the protection demonstrated by vaccination with a monovalent anthrax RP vaccine (Lee et al., 2003). Hooper et al. combined individual RP expressing four different smallpox virus antigens and demonstrated protection from lethal monkeypox virus challenge in cynomolgus macaques (Hooper et al., 2009). Taken together, these results indicate that multivalent RP vaccines, regardless of the production method, are able to induce balanced antigenspecific immune responses and can elicit protection against multi-agent challenge. These results have important implications in decreasing the number of injections required for protection against multiple diseases or serotypes of a single disease agent and also for decreasing the cost of RP vaccine production.
Resistance to anti-vector immunity
As the alphavirus structural genes are not packaged and thus not expressed following vaccination, anti-vector immune responses are minimal. This lack of anti-vector immunity allows for multiple vaccinations of the same individual with either the same RP vaccine or different RP vaccines against multiple pathogens. Pushko et al. first demonstrated that RP could be used for sequential immunization by vaccinating mice with two doses of RP expressing the N protein of Lassa virus and then sequentially vaccinating the same mice with two doses of HA RP (Pushko et al., 1997). These mice developed positive serum antibody responses against both antigens. Additionally, vaccinated mice were protected against influenza challenge, indicating that the Lassa N immune response did not interfere with subsequent influenza vaccination and the influenza-specific immune response. Similar results have been achieved following vaccination with RP expressing the HA protein from two different subtypes of influenza (our unpublished results). Ferrets were first immunized with an H3 RP vaccine and subsequently protected against homologous H3N2 influenza challenge. These protected ferrets (pre-immunized) were then vaccinated with H1 RP along with a group of nai¨ve (non-H3 RP vaccinated) animals. Both the pre-immunized and nai¨ve ferrets that received the H1 RP vaccine developed equivalent H1N1 hemagglutination inhibition (HI) serum antibody titers. These studies confirm that RP vaccines can be used sequentially without an inhibitory effect on vaccine efficacy. Other recombinant vaccines are often hindered by anti-vector immunity and it has been suggested that recombinant adenovirus vaccination regimens should include two heterologous vectors to avoid anti-vector immunity (Thorner et al., 2006).
Commercial vaccine production
Many diseases that have not had efficacious vaccines developed against them are in the NIH Risk Group 3 (such as highly pathogenic avian influenza H5N1 and human immunodeficiency virus (HIV) types 1 and 2) or on the APHIS/CDC Select Agent List (such as botulinum neurotoxins, Ebola and Marburg viruses, B. anthracis, Hendra and Nipah viruses, foot-and-mouth disease virus (FMDV) and CSFV). Thus, all of these pathogens require either BSL3 or BSL4 production facilities for traditional vaccine production. This requirement has almost certainly been an impediment in the development of some of these vaccines. In addition, the alphavirus VEEV is listed as a select agent, except for the TC-83 attenuated vaccine strain. Thus, the TC-83 infectious clone can be utilized in the replicon system expressing the protective genes of the aforementioned select agents at low biocontainment levels for research and production. Since replicon-based vaccine production does not require growth of the pathogenic organism, select agent replicon-based vaccine development and production can occur in low biocontainment production facilities with no special biosecurity required. The capability to produce select agent vaccines in such production facilities is a huge advantage of the replicon system and has aided the development of these crucial vaccines for both humans and animals.
The attenuating determinants of TC-83 virus attenuation have been well defined (Kinney et al., 1988, 1993). Because of the safety profile of attenuated TC-83, it has been recently developed as a replicon vector (Kamrud et al., 2008; Hooper et al., 2009; Bosworth et al., 2010; Erdman et al., 2010; Vander Veen et al., 2012). A recent study compared a TC-83-based RP vaccine against a different RP vaccine (V3014-based vaccine produced in BSL3 facilities) in pigs. Both RP vaccines expressing H3N2 HA elicited the same antibody response in pigs, demonstrating that there is no difference in immunogenicity between RP vaccines produced using these two VEEV replicon systems (Erdman et al., 2010).
Another advantage of RP vaccines is that many different cell lines are permissive to alphavirus infection, allowing a variety of cells to be evaluated for maximum yields. Vero and baby hamster kidney (BHK) cells are most commonly used for RP production, but Chinese hamster ovary (CHO), primary chicken embryo fibroblasts (CEF) and duck embryo fibroblasts (DEF), human embryonic kidney 293 and 293 T cell lines have also been utilized for RP production (our unpublished results; Pushko et al., 1997). This long list of cells is in contrast to that for pathogenic virus growth which usually occurs optimally in one cell line. As in traditional vaccine production, these cells can be grown in large quantities using large-scale bioreactor microcarrier or suspension systems allowing efficient scale-up possibilities for RP production. There is also a report of stably transfected cell lines that have been developed to constitutively express the helper RNAs needed for RP production (Polo et al., 1999). Similar to the split helper RNA system described above, only cell lines containing the structural protein genes on separate RNAs resulted in no recovery of RCV (Polo et al., 1999).
DIVA capability
One of the attributes of a good next-generation vaccine is the capability to permit DIVA. The DIVA concept relies on the principle that a vaccinated animal will have a different immune response than an animal that is infected with the wild-type pathogen and that this immune response is readily detectable by some immunoassay. These DIVA vaccines become increasingly important when considering diseases that are not currently present in disease-free status countries (such as FMDV and CSFV) or for disease eradication and intense surveillance programs. In the case of FMDV, the current control policy has been primarily one of slaughtering the infected and contact animals (Uttenthal et al., 2010). This policy could have a huge detrimental impact on domestic livestock production and potential export ramifications. Following the 2001 FMDV outbreak in the UK, there has been a growing demand for FMDV vaccination following an outbreak in order to reduce the large-scale slaughter of animals for control of the virus (Uttenthal et al., 2010). Current FMDV vaccine research is focused on development of DIVA vaccines expressing the capsid proteins, including FMDV repliconbased vaccine research (our unpublished results; Yu et al., 2006; Meeusen et al., 2007).
Vaccines that have DIVA capability are also important for the control and eventual eradication of current endemic infectious diseases. PRRSV is endemic to the United States swine population and continues to have a huge economic impact (Neumann et al., 2005). Current diagnostic assays for PRRSV target antibodies directed toward the nucleocapsid (N) protein. Antibodies to the N protein have been shown to be non-neutralizing (Dea et al., 2000), therefore a vaccine need not include this protein to be efficacious. Thus, RP vaccines expressing any combination of PRRSV structural glycoproteins would therefore permit the differentiation of vaccinated from naturally infected animals. Similarly, a current ELISA for detecting influenza in pigs is based on NP antibody detection (Ciacci-Zanella et al., 2010). RP vaccines expressing only the HA protein would therefore not induce a detectable immune response when used in conjunction with this diagnostic assay. Therefore, alphavirus replicon-based vaccines offer DIVA capabilities that can be important in different disease situations.
Conclusions
Research into the potential of alphavirus replicon-based vaccines has been ongoing for more than 20 years. Significant advancements have been made since these vectors were first used for the expression of heterologous genes. Improvements in both safety and the replicon vector design have significantly advanced the field of replicon-based vaccines. Both RP and replicon DNA vaccines have demonstrated robust and balanced immune responses with subsequent protection against a variety of diseases that have implications for both veterinary and human health. Thus, the alphavirus replicon technology offers great potential for the next generation of animal and human vaccines.
References
Alevizatos AC, McKinney RW and Feigin RD (1967). Live, attenuated Venezuelan equine encephalomyelitis virus vaccine: clinical effects in man. American Journal of Tropical Medicine and Hygiene 16: 762–768.
Altman-Hamamdzic S, Groseclose C, Ma JX, Hamamdzic D, Vrindavanam NS, Middaugh LD, Parratto NP and Sallee FR (1997). Expression of beta-galactosidase in mouse brain: utilization of a novel nonreplicative Sindbis virus vector as a neuronal gene delivery system. Gene Therapy 4: 815–822.
Balasuriya UBR, Heidner HW, Davis NL, Wagner HM, Hullinger PJ, Hedges JF, Williams JC, Johnston RE, Wilson WD, Liu IK and Maclachlan NJ (2002). Alphavirus replicon particles expressing the two major envelope proteins of equine arteritis virus induce high level protection against challenge with virulent virus in vaccinated horses. Vaccine 20: 1609–1617.
Balasuriya UBR, Heidner HW, Hedges JF, Williams JQ, Davis NL, Johnston RE and Maclachlan NJ (2000). Expression of the two major envelope proteins of equine arteritis virus as a heterodimer is necessary for induction of neutralizing antibodies in mice immunized with recombinant Venezuelan equine encephalitis virus replicon particles. Journal of Virology 74: 10623–10630.
Berglund P, Sjoberg M, Garoff H, Atkins GJ, Sheahan BJ and Liljestrom P (1993). Semliki Forest virus expression system: production of conditionally infectious recombinant particles. Biotechnology (NY) 11: 916–920.
Berglund P, Smerdou C, Flee MN, Tubulekas I and Liljestrom P (1998). Enhancing immune responses using suicidal DNA vaccines. Nature Biotechnology 16: 562–565.
Bernstein DI, Reap EI, Katen K, Watson A, Smith K, Norberg P, Olmsted RA, Hoeper A, Morris J, Negri S, Maughan MF and Chulay JD (2010). Randomized, double-blind, phase 1 trial of an alphavirus replicon vaccine for cytomegalovirus in CMV seronegative adult volunteers. Vaccine 28: 484–493.
Bosworth B, Erdman MM, Stine DL, Harris I, Irwin C, Jens M, Loynachan A, Kamrud K and Harris DL (2010). Replicon particle vaccine protects swine against influenza. Comparative Immunology, Microbiology, and Infectious Diseases 33: e99–e103.
Botner A, Strandbygaard B, Sorensen KJ, Have P, Madsen KG, Madsen ES and Alexandersen S (1997). Appearance of acute PRRS-like symptoms in sow herds after vaccination with a modified live PRRS vaccine. Veterinary Record 141: 497–499.
Bredenbeek PJ, Frolov I, Rice CM and Schlesinger S (1993). Sindbis virus expression vectors: packaging of RNA replicons by using defective helper RNAs. Journal of Virology 67: 6439–6446.
Burke DS and Ramsburg HH (1977). Persistence in humans of antibody to subtypes of Venezuelan equine encephalomyelitis (VEE) virus after immunization with attenuated (TC-83) VEE virus vaccine. Journal of Infectious Diseases 136: 354–359.
Carrol TD, Matzinger SR, Barro M, Fritts L, McChesney MB, Miller CJ and Johnston RE (2011). Alphavirus repliconbased adjuvants enhance the immunogenicity and effectiveness of Fluzone in rhesus macaques. Vaccine 29: 931–940.
Ciacci-Zanella JR, Vincent AL, Prickett JR, Zimmerman SM and Zimmerman JJ (2010). Detection of anti-influenza A nucleoprotein antibodies in pigs using a commercial influenza epitope-blocking enzyme-linked immunosorbent assay developed for avian species. Journal of Veterinary Diagnostic Investigation 22: 3–9.
Dea S, Gagnon CA, Mardassi H, Pirzadeh B and Rogan D (2000). Current knowledge on the structural proteins of porcine reproductive and respiratory syndrome (PRRS) virus: comparison of the North American and European isolates. Archives of Virology 145: 659–688.
Defang GN, Khetawat D, Broder CC and Quinnan Jr GV (2011). Induction of neutralizing antibodies to Hendra and Nipah glycoproteins using a Venezuelan equine encephalitis virus in vivo expression system. Vaccine 29: 212–220.
Dubensky Jr TW, Driver DA, Polo JM, Belli BA, Latham EM, Ibanez CE, Chada S, Brumm D, Banks TA, Mento SJ, Jolly DJ and Chang SM (1996). Sindbis virus DNA-based expression vectors: utility for in vitro and in vivo gene transfer. Journal of Virology 70: 508–519.
Erdman MM, Kamrud KI, Harris DL and Smith J (2010). Alphavirus eplicon particle vaccines developed for use in humans induce high levels of antibodies to influenza virus hemagglutinin in swine: proof of concept. Vaccine 28: 594–596.
Frolov I, Frolova E and Schlesinger S (1997). Sindbis virus replicons and Sindbis virus: assembly of chimeras and of particles deficient in virus RNA. Journal of Virology 71: 2819–2829.
Gardner JP, Frolov I, Perri S, Ji Y, MacKichan ML, Zur Megede J, Chen M, Belli BA, Driver DA, Sherrill S, Greer CD, Olten GR, Barnett SW, Liu MA, Dubensky TW and Polo JM (2000). Infection of human dendritic cells by a Sindbis viral replicon vector is determined by a single amino acid substitution in the E2 glycoprotein. Journal of Virology 74: 11849–11857.
Geigenmuller-Gnirke U, Weiss B, Wright R and Schlesinger S (1991). Complementation between Sindbis viral RNAs produces infectious particles with a bipartite genome. Proceedings of the National Academy of Sciences of the United States of America 88: 3253–3257.
Griffin DE (2007). Alphaviruses. In: Knipe DM and Howley PM (eds) Fields Virology, 5th edn Lippincott Williams & Wilkins, pp. 1023–1067.
Hariharan MJ, Driver DA, Townsend K, Brumm D, Polo JM, Belle BA, Catton DJ, Hsu D, Mittelstaedt D, McCormack JE, Karavodin L, Dubensky Jr DW, Chang SM and Banks TA (1998). DNA immunization against herpes simplex virus: enhanced efficacy using a Sindbis virus-based vector. Journal of Virology 72: 950–958.
Hooper JW, Ferro AM, Golden JW, Sivera P, Dudek JM, Alterson KD, Custer M, Rivers B, Morris J, Owens G, Smith JF and Kamrud KI (2009). Molecular smallpox vaccine delivered by alphavirus replicons elicits protective immunity in mice and non-human primates. Vaccine 28: 494–511.
Hubby B, Talarico T, Maughan M, Reap EA, Berglund P, Kamrud KI, Copp L, Lewis W, Cecil C, Norberg P, Wagner J, Watson A, Negri S, Burnett BK, Graham A, Smith JF and Chulay JD (2007). Development and preclinical evaluation of an alphavirus replicon vaccine for influenza. Vaccine 25: 8180–8189.
Jiang W, Jiang P, Li Y, Tang J, Wang X and Ma S (2006a). Recombinant adenovirus expressing GP5 and M fusion proteins of porcine reproductive and respiratory syndrome virus induce both humoral and cell-mediated responses in mice. Veterinary Immunology and Immunopathology 113: 169–180.
Jiang Y, Fang L, Xiao S, Zhang H, Pan Y, Luo R, Li B and Chen H (2006b). Immunogenicity and protective efficacy of recombinant pseudorabies virus expressing the two major membrane-associated proteins of porcine reproductive and respiratory syndrome virus. Vaccine 25: 547–560.
Jiang Y, Xiao S, Fang L, Yu X, Song Y, Niu C and Chen H (2006c). DNA vaccines co-expressing GP5 and M proteins of porcine reproductive and respiratory syndrome virus (PRRSV) display enhanced immunogenicity. Vaccine 24: 2869–2879.
Johnston LJ, Halliday GM and King NJ (2000). Langerhans cells migrate to local lymph nodes following cutaneous infection with an arbovirus. Journal of Investigative Dermatology 114: 560–568.
Jose J, Snyder JE and Kuhn RJ (2009). A structural and functional perspective of alphavirus replication and assembly. Future Microbiology 4: 837–856.
Kamrud KI, Alterson K, Custer M, Dudek J, Goodman C, Owens G and Smith JF (2010a). Development and characterization of promotorless helper RNAs for the production of alphavirus replicon particles. Journal of General Virology 91: 1723–1727.
Kamrud KI, Alterson KD, Andrews C, Copp LO, Lewis WC, Hubby B, Patel D, Rayner JO, Talarico T and Smith JF (2008). Analysis of Venezuelan equine encephalitis replicon particles packaged in different coats. PLoS ONE 3: 1–8.
Kamrud KI, Coffield VM, Owens G, Goodman C, Alterson K, Custer M, Lewis W, Timberlake S, Wansley EK and Berglund P (2010b). In vitro and in vivo characterization of microRNA-targeted alphavirus replicon and helper RNAs. Journal of Virology 84: 7713–7725.
Kamrud KI, Custer M, Dudek JM, Owens G, Alterson KD, Lee JS, Groebner JL and Smith JF (2007). Alphavirus replicon approach to promoterless analysis of IRES elements. Virology 360: 376–387.
Kinney RM, Chang GJ, Tsuchiya KR, Sneider JM, Roehrig JT, Woodward TM and Trent DW (1993). Attenuation of Venezuelan equine encephalitis virus strain TC-83 is encoded by the 50-noncoding region and the E2 envelope glycoprotein. Journal of Virology 67: 1269–1277.
Kinney RM, Johnson BJB, Welch JB, Tsuchiya KR and Trent DW (1988). The full-length nucleotide sequences of the virulent Trinidad donkey strain of Venezuelan equine encephalitis virus and its attenuated vaccine derivative, strain TC-83. Virology 170: 19–30.
Kohno A, Emi N, Kasai M, Tanimoto M and Saito H (1998). Semliki Forest virus-based DNA expression vector: transient protein production followed by cell death. Gene Therapy 5: 415–418.
Kowalski J, Adkins K, Gangolli S, Ren J, Arendt H, DeStefano J, Obregon J, Tummolo D, Natuk R, Brown T, Parks C, Udem S and Long D (2007). Evaluation of neurovirulence and biodistribution of Venezuelan equine encephalitis replicon particles expressing herpes simplex virus type 2 glycoprotein D. Vaccine 25: 2296–2305.
Lee JS, Groebner JL, Hadjipanayis AG, Negley DL, Schmaljohn AL, Welkos SL, Smith LA and Smith JF (2006). Multiagent vaccines vectored by Venezuelan equine encephalitis virus replicon elicits immune responses to Marburg virus and protection against anthrax and botulinum neurotoxin in mice. Vaccine 24: 6886–6892.
Lee JS, Hadjipanayis AG and Welkos SL (2003). Venezuelan equine encephalitis virus-vectored vaccines protect mice against anthrax spore challenge. Infection and Immunity 71: 1491–1496.
Levinson R, Strauss JH and Strauss EG (1990). Determination of the complete nucleotide sequence of the genomic RNA of the O'nyong-nyong virus and its use in the construction of phylogenetic trees. Virology 175: 110–123.
Li N, Zhao JJ, Zhao HP, Sun Y, Zhu QH, Tong GZ and Qiu HJ (2007). Protection of pigs from lethal challenge by a DNA vaccine based on an alphavirus replicon expressing the E2 glycoprotein of classical swine fever virus. Journal of Virological Methods 144: 73–78.
Liljestrom P and Garoff H (1991). A new generation of animal cell expression vectors based on the Semliki Forest virus replicon. Biotechnology (NY) 9: 1356–1361.
Ljungberg K, Whitmore AC, Fluet ME, Moran TP, Shabman RS, Collier ML, Kraus AA, Thompson JM, Montefiori DC, Beard C and Johnston RE (2007). Increased immunogenicity of a DNA-launched Venezuelan equine encephalitis virusbased replicon DNA vaccine. Journal of Virology 81: 13412–13423.
Lundstrom K, Grayson RJ, Richard PJ and Francois J (1999). Efficient in vivo expression of a reporter gene in a rat brain after injection of recombinant replication-deficient Semliki Forest virus. Gene Therapy and Molecular Biology 3: 15–23.
MacDonald GH and Johnston RE (2000). Role of dendritic cell targeting in Venezuelan equine encephalitis virus pathogenesis. Journal of Virology 74: 914–922.
Madsen KG, Hansen CM, Madsen ES, Strandbygaard B, Botner A and Sorensen KJ (1998). Sequence analysis of porcine reproductive and respiratory syndrome virus of the American type collected from Danish swine herds. Archives in Virology 143: 1683–1700.
Meeusen ENT, Walker J, Peters A, Pastoret PP and Jungersen G (2007). Current status of veterinary vaccines. Clinical Microbiology Reviews 20: 489–510.
Minke JM, Audonnet JC and Fischer L (2004). Equine viral vaccines: the past, present and future. Veterinary Research 35: 425–443.
Mogler M (2009). Replicon particle PRRSV vaccine provides partial protection from challenge. Proceedings of the American Association of Swine Veterinarians 2009: 367–368.
Mogler M, Vander Veen R, McVicker J, Russell B and Harris DL (2010). Vaccination of pigs with PRRVENT or PRRSV-RP recombinant vaccines reduces viremia following heterologous challenge. Proceedings of the American Association of Swine Veterinarians 2010: 403–404.
Morris-Downes MM, Phenix KV, Smyth J, Sheahan BJ, Lilegvist S, Mooney DA, Liljestrom P, Todd D and Atkins GJ (2001). Semliki Forest virus-based vaccines: persistence, distribution and pathological analysis in two animal systems. Vaccine 19: 1978–1988.
Neumann EJ, Kleibenstein J, Johnson C, Mabry JW, Bush EJ, Seitzinger AH, Green AL and Zimmerman JJ (2005). Assessment of the economic impact of porcine reproductive and respiratory syndrome on swine production in the United States. Journal of the American Veterinary Medical Association 227: 385–392.
Nishimoto KP, Laust AK, Wang K, Kamrud KI, Hubby B, Smith JF and Nelson EL (2007). Restricted and selective tropism of a Venezuelan equine encephalitis virus-derived replicon vector for human dendritic cells. Viral Immunology 20: 88–104.
Pittman PR, Makuch RS, Mangiafico JA, Cannon TL, Gibbs PH and Peters CJ (1996). Long-term duration of detectable neutralizing antibodies after administration of live-attenuated VEE vaccine and following booster vaccination with inactivated VEE vaccine. Vaccine 14: 337–343.
Polo JM, Belli BA, Driver DA, Frolov I, Sherrill S, Hariharan MJ, Townsend K, Perri S, Mento SJ, Jolly DJ, Chang SMW, Schlesinger S and Dubensky Jr TW (1999). Stable alphavirus packaging cell lines for Sindbis virus- and Semliki Forest virus-derived vectors. Proceedings of the National Academy of Sciences of the United States of America 96: 4598–4603.
Pushko P, Geisbert J, Parker M, Jahrling P and Smith J (2001). Individual and bivalent vaccines based on alphavirus replicons protect guinea pigs against infection with Lassa and Ebola viruses. Journal of Virology 75: 11677–11685.
Pushko P, Parker M, Ludwig GV, Davis NL, Johnston RE and Smith JF (1997). Replicon-helper systems from attenuated Venezuelan equine encephalitis virus: expression of heterologous genes in vitro and immunization against heterologous pathogens in vivo. Virology 239: 389–401.
Rayner JO, Dryga SA and Kamrud KI (2002). Alphavirus vectors and vaccination. Reviews in Medical Virology 12: 279–296.
Reap EA, Dryga SA, Morris J, Rivers B, Norberg PK, Olmsted RA and Chulay JD (2007a). Cellular and humoral immune responses to alphavirus replicon vaccines expressing cytomegalovirus pp65, IE1, and gB proteins. Clinical and Vaccine Immunology 14: 748–755.
Reap EA, Morris J, Dryga SA, Maughan M, Talarico T, Esch RE, Negri S, Burnett B, Graham A, Olmsted RA and Chulay JD (2007b). Development and preclinical evaluation of an alphavirus replicon particle vaccine for cytomegalovirus. Vaccine 25: 7441–7449.
Saxena S, Dahiya SS, Sonwane AA, Patel CL, Saini M, Rai A and Gupta PK (2008). A Sindbis virus replicon-based DNA vaccine encoding the rabies virus glycoprotein elicits immune responses and complete protection in mice from lethal challenge. Vaccine 26: 6592–6601.
Schultz-Cherry S, Dybing JK, Davis NL, Williamson C, Suarez DL, Johnston R and Perdue ML (2000). Influenza virus (A/HK/ 156/97) hemagglutinin expressed by an alphavirus replicon system protects chickens against lethal Infection with Hong Kong-origin H5N1 viruses. Virology 278: 55–59.
Smerdou C and Liljestrom P (1999). Two-helper RNA system for production of recombinant Semliki Forest virus particles. Journal of Virology 73: 1092–1098. Strauss J and Strauss E (1994). The alphaviruses: gene expression, replication, and evolution. Microbiological Reviews 58: 491–562.
Sun SQ, Liu T, Guo HC, Yin SH, Shang YJ, Feng X, Liu ZX and Xie QG (2007). Protective immune responses in guinea pigs and swine induced by a suicidal DNA vaccine of the capsid gene of swine vesicular disease virus. Journal of General Virology 88: 842–848.
Sylte MJ, Hubby B and Suarez DL (2007). Influenza neuraminidase antibodies provide partial protection for chickens against high pathogenic avian influenza infection. Vaccine 25: 3769–3772.
Takkinen K (1986). Complete nucleotide sequence of the nonstructural protein genes of Semliki Forest virus. Nucleic Acids Research 14: 5667–5682.
Thorner AR, Lemckert AAC, Goudsmit J, Lynch DM, Ewald BA, Denholtz M, Havenga MJE and Barouch DH (2006). Immunogenicity of heterologous recombinant adenovirus prime-boost vaccine regimens is enhanced by circumventing vector cross-reactivity. Journal of Virology 80: 12009–12016.
Uttenthal A, Parida S, Rasmussen TB, Paton DJ, Haas B and Dundon WG (2010). Strategies for differentiating infection in vaccinated animals (DIVA) for foot-and-mouth disease, classical swine fever and avian influenza. Expert Reviews of Vaccines 9: 73–87.
Vander Veen RL, Kamrud KI, Mogler MA, Loynachan AT, McVicker J, Owens G, Berglund P, Timberlake S, Whitney L, Smith J and Harris DL (2009). Rapid development of an efficacious swine vaccine for novel H1N1. PLoS Currents Influenza 1: RRN1123.
Vander Veen RL, Loynachan AT, Mogler MA, Russell BJ, Harris DL and Kamrud KI (2012). Safety, immunogenicity, and efficacy of an alphavirus-based swine influenza virus hemagglutinin vaccine. Vaccine 11: 1944–1950.
Volkova E, Gorchakov R and Frolov I (2006). The efficient packaging of Venezuelan equine encephalitis virus-specific RNAs into viral particles is determined by nsp1–3 synthesis. Virology 344: 315–327.
Weiss BG and Schlesinger S (1991). Recombination between Sindbis virus RNAs. Journal of Virology 65: 4017–4025.
Yu X, Xiao S, Fang L, Jiang Y and Chen H (2006). Enhanced immunogenicity to foot-and-mouth disease virus in mice following vaccination with alphaviral replicon-based DNA vaccine expressing the capsid precursor polypeptide (P1). Virus Genes 33: 337–344.
Zhao HP, Li N, Sun Y, Wang Y and Qiu HJ (2009a). Prime-boost immunization using alphavirus replicon and adenovirus vectored vaccines induces enhanced immune responses against classical swine fever virus in mice. Veterinary Immunology and Immunopathology 15: 158–166.
Zhao HP, Sun JF, Li N, Sun Y, Xia ZH, Wang Y, Cheng D, Qi F, Jin ML and Qiu HJ (2009b). Assessment of the cell-mediated immunity induced by alphavirus replicon-vectored DNA vaccines against classical swine fever in a mouse model. Veterinary Immunology and Immunopathology 129: 57–65.