Introduction
In non-ruminants, the peroxisome proliferator-activated receptors (PPAR) play a crucial role in the regulation of fatty acid metabolism and inflammatory responses [1]. The PPAR family is composed of three subtypes: PPAR-gamma (PPARG), PPAR-alpha (PPARA), and PPARδ/β (PPARD), among which the expression of PPARG and PPARA subtypes are associated with adipogenesis/lipogenesis and catabolism of fatty acids, respectively [1]. A comprehensive review in ruminants underscored the potential for manipulating PPAR to fine-tune metabolism and immune function during growth and lactation [2]. Because PPARG plays a pivotal role in adipogenesis of adipose tissue (AT), its regulation through insulin-sensitizing agonists, nutrition, and obesity in dairy cows could have important implications for the control of energy homeostasis, particularly during the period between late pregnancy and early lactation, which is characterized by marked AT lipolysis due in part to a reduction in insulin concentration. This is one of several adaptations in dairy cows to prioritize the use of nutrients for mammary synthesis of milk.
Thiazolidinediones are potent insulin-sensitizing agonists of the nuclear receptor PPARG [3]. The results of previous studies with dairy cows underscored the possibility that 2,4-Thiazolidinedione (TZD) can ameliorate insulin insensitivity, likely via PPARG [4–6]. The administration of TZD (4 mg of TZD/kg of BW) during the late prepartum period appeared to alter the dynamics of plasma glucose, non-esterified fatty acids (NEFA), and beta-hydroxybutyrate (BHBA) concentrations as well as dry matter intake (DMI) during the periparturient period, and helped cows maintain body condition score (BCS) postpartum [4, 7]. Furthermore, a greater concentration of insulin in blood was observed in TZD-treated cows, which likely accounted for the lower NEFA [6]. In humans, the mean clearance rate (i.e. change in plasma concentration over time) of rosiglitazone, a TZD, was reported to be lower in female compared with male patients of the same body weight [8]. However, no such data exist for dairy cow but could be key for understanding temporal changes in profiles of PPAR-related and insulin-sensitivity related genes [2].
Our hypothesis was that plane of nutrition leads to changes in BW and insulin sensitivity in subcutaneous AT (SAT) and skeletal muscle tissue. Furthermore, the peripheral and systemic insulin-sensitizing effect of TZD occurs at least in part via molecular alterations in PPAR signaling networks. Therefore, the objective of the current study was to evaluate the effect of plane of energy nutrition and TZD administration on energy intake (NEL), body mass, body fatness, energy balance markers (e.g. NEFA, BHBA, and glucose), insulin, and the insulin-sensitizing hormone adiponectin (ADIPOQ) in non-pregnant and non-lactating dairy cows.
Materials and Methods
Experimental Design, Diet and Animal Management
The Institutional Animal Care and Use Committee (IACUC) of the University of Illinois approved all procedures for this study (protocol #12134). Fourteen nonlactating non-pregnant Holstein cows [initial body weight (BW, kg) = 717 ±39; initial BCS = 3.31 ± 0.14] were assigned randomly into the treatment groups. Cows were offered the TMR once daily at 0600 h and had unlimited access to fresh water. All cows were fed a control diet (low-energy, CON; NEL = 1.30 Mcal/kg) to meet 100% of National Research Council (NRC) requirements at ad libitum DMI for 3 weeks, after which half of the cows were assigned to a higher-energy diet (OVE; NEL = 1.60 Mcal/kg) and half of the cows continued on CON for 6 weeks. The OVE diet was fed ad libitum and resulted in cows consuming ~180% of NRC requirements. Control cows were fed to consume only 100% of NRC. The ingredient and nutrient composition of both diets are presented in Table 1. All cows received an intravenous injection of 4 mg TZD/kg of BW daily into the jugular vein starting 2 weeks after the initiation of treatments and for 2 additional weeks. The last 2 weeks of the study served as the washout period. Cows were housed in ventilated indoor pens (10 m × 15 m; photoperiod of 8 h light and 16 h dark) equipped with individual electronic transmission gates and transponders (American Calan, Northwood, NH) for access to feed. Each pen had 10 sand-bedded free stalls with at least one stall per cow.
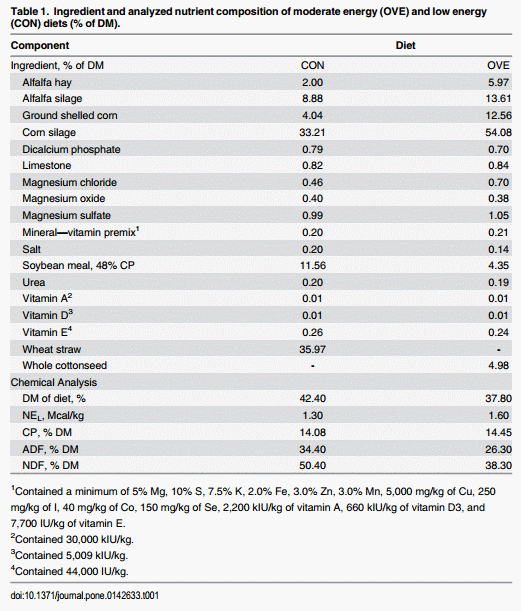
Sample Collection Blood samples were collected before the morning feeding from the coccygeal vein or artery every 5 d ± 2 from –7 to 14 d relative to diet initiation, before TZD administration or from 15 to 28 d relative to diet initiation and during TZD administration for measurement of metabolites and hormones. Samples were collected into evacuated tubes (Vacutainer, BD and Co., Franklin Lakes, NJ) containing clot activator or lithium heparin. After blood collection, tubes containing lithium heparin were placed on ice, while the tubes with clot activator were kept ~ 30 min at 21°C until centrifugation. Serum and plasma were obtained by centrifugation of clot activator and lithium heparin tubes, respectively, at 1,900 × g for 15 min and frozen at −80°C until later analysis.
Blood Metabolites and Hormones. Blood metabolites were analyzed in lithium heparin samples at 37°C following the procedures previously described by Bionaz et al. [9] in a clinical auto-analyzer (ILAB 600, Instrumentation Laboratory, Lexington, MA, USA). Glucose, NEFA and BHBA were determined using commercial kits purchased from Instrumentation Laboratory (IL Test), Wako Chemicals GmbH (Neuss, Germany) and Randox Laboratories Ltd. (Crumlin, Co. Antrium, UK) respectively. Adiponectin was measured in serum samples using the ELISA assay developed by Mielenz et al. [10], while insulin was assayed by a double-antibody radioimmunoassay (RIA) using a primary antiserum to bovine insulin as described by Sosa et al. [11]. The ratios of NEFA and glucose to insulin were calculated to estimate systemic insulin sensitivity.
Tissue biopsies, RNA Isolation, Primer Design and Evaluation, and Quantitative PCR
Subcutaneous AT and skeletal muscle biopsies were collected from alternate sides before the morning feeding at 14, 21 and 28 d, and 35 d relative to diet initiation. The TZD was injected starting on d 14 and continued for 2 additional weeks. Details of these procedures are reported in the supporting information. The selection of the primers for SAT was conducted based on core cellular functions, e.g. insulin signaling and responsiveness, adipogenic and lipogenic enzymes/inducers, and post-translational modifiers; while the focus on skeletal muscle tissue was based on core cellular functions, e.g. lipid and carbohydrate biosynthesis and metabolism (Table A and Table B in S1 File). The amplicons were sequenced and the fragment sequences were blasted and confirmed using the National Center for Biotechnology Information (NCBI). The sequences are presented in the supporting information http://www.journalofdairyscience.org/. The geometric mean of the internal control (ICG) genes GAPDH, ribosomal protein S9 (RPS9), and ubiquitously-expressed transcript (UXT) were used for data normalization (V2/3 ≤0.15) in SAT, while the geometric mean of reference genes mitochondrial ribosome-associated GTPase 1 (MTG1), ribosomal protein S15A (RPS15A) and UXT were used for data normalization (V2/3 ≤0.15) in skeletal muscle tissue.
Muscle Triacylglycerol Concentration
A total of 50 mg of tissue was first homogenized in 1.5 mL of PBS/10 mM EDTA using a handheld homogenizer (Tissue-Tearor, Biospec Products). Subsequently, 200 μL of GPBS-EDTA along with 3 mL of isopropanol-hexane-water (80:20:2 vol/vol) were added to each sample and the mixture incubated covered with aluminum foil for 30 min at room temperature. One mL of hexane-diethyl ether (1:1) was then added to each sample followed by vortexing and incubating for 10 min at room temperature (protected from light). One mL of water was added to each sample to separate the lipid phase and the mixture was vortexed. Samples were incubated covered with aluminum foil for ~20 min at room temperature. The organic phase was then aspirated and placed into glass vials, prior to evaporation under a stream of N gas. An 8-point TAG standard was prepared with Infinity TG reagent (cat#10010509, Cayman Chemicals). Each sample was mixed with 540 μL of Infinity TG reagent prior to vortexing. A total of 160 μL of this sample mixture was pipetted into a flat-bottom 96-well plastic microplate. The plate was incubated for 15 min at 37°C prior to determining absorbance at 540 nm using a microplate reader. Concentration of TAG was calculated from the standard curve. The final concentration is expressed as mg TAG/g of tissue.
Western Blot Analysis
The entire procedure is reported in the supporting information. Briefly, Western blotting was conducted to verify a subset of the key changes observed in protein expression of PPARG in SAT. The SAT was homogenized and a hundred micrograms of total protein were subjected to 12% SDS/PAGE and transferred to a PVDF membrane (Millipore). The membrane was probed first with rabbit anti- PPARG antibody (Abcam, Cambridge, MA) and rabbit anti-GAPDH antibody (Abcam). The secondary antibody was goat polyclonal secondary antibody to rabbit IgG—H&L (HRP) (Abcam). Immunodetection using the Clarity Western ECL Substrate (Biorad, Hercules, CA) was performed according to the manufacturer’s instruction. The intensities of the bands were quantified [12, 13] using ImageJ software [14] and the expression of PPARG protein was normalized to the expression level of GAPDH protein.
TZD Measurement in Serum
Two hundred μL of serum was precipitated by addition of 200 μL of ethyl acetate in 2 mL micro centrifuge tubes. The tubes were vortexed for 2 min and centrifuged at 10,000 g for 10 min. The supernatant was transferred to a clean tube. All extracts were evaporated under nitrogen at room temperature. Then they were reconstituted with 100 μL of ethanol [15]. The HPLC system included a Hewllett Packard 1100 System with diode array detector. Separation were performed on a Hypersil BDS C-18 (250 mm x 4.6 mm i.d., 5 μm particle size) (Thermo Scientific, Waltham, MA) analytical column. The TZD was eluted isocratically with methanol- 20 mM MOPS buffer at pH 7.4 (80:20, v/v) at a flow rate of 1 mL min-1, with TZD detection at 230 nm. In all cases, a 20 μL aliquot was injected by duplicate into the HPLC system [16].
Gene Network Analysis
Gene network analysis was performed using Ingenuity Pathway Analysis1 (IPA; http://www. ingenuity.com, Redwood City, CA). Only direct interactions among the genes analyzed in the present study were included in the analyses. The IPA database considers only the known interactions in published studies with humans and rodents. The data obtained on biopsies harvested at 14 d of diet initiation were considered as the reference point to generate the IPA input data as fold-change. Gene networks generated from the analysis can be found in the S1 File of the Supporting Information material.
Statistical Analysis
All data were analyzed with the Proc MIXED procedure of SAS 9.4 (SAS Institute Inc., Cary, NC). After normalization with the geometric mean of the ICG, the triplicate qPCR data for each gene were averaged and then log2 transformed prior to statistical analysis. Fixed effects in the model were diet, time, and diet × time. Cow within diet was designated as the random effect. Initial BW and BCS were included as covariates in the analysis for all variables, except when the covariate was not significant (P > 0.05). The Kenward-Roger statement was used for computing the denominator degrees of freedom, while spatial power was used as the covariance structure. The same statistical model was used for the protein data. Spearman rank correlation coefficients were derived to identify possible relationships among the interval-measured blood variables. Significance was declared at P ≤ 0.05, while trends were declared at P ≤ 0.10 for the gene expression data.
Results
Energy Intake and Body Mass
Cows fed OVE had greater NEL intake (P < 0.05; 19.91 vs. 10.58 Mcal/d; diet × day P < 0.05; Fig 1) than CON cows. Body weight was greater (diet × day P < 0.05; Fig 1) in OVE compared with CON cows at 2 to 6 weeks after diet initiation. The NEL intake and BW were affected by the interaction of diet × day (P < 0.05) because of the gradual increase of DMI in OVE cows compared with the constant NEL intake in CON cows. The TZD administration did not affect NEL intake and BW (Fig 1) but OVE cows had greater energy intake (P ≤ 0.05) during the washout period compared with the TZD administration period at 28 d.
Blood Metabolites and Hormones before TZD Administration
Main effects of diet, time, and their interactions for biomarkers are reported in Fig 1. The OVE cows had lower concentrations of NEFA (diet × day, P ≤ 0.05; Fig 1) and ADIPOQ (diet × day, P ≤ 0.05; Fig 1) compared with CON. There was an increase (diet × day, P ≤ 0.05; Fig 1) in the concentration of insulin in OVE cows at 2 weeks after diet initiation, while there was no effect on glucose and BHBA. The ratio of glucose and NEFA to insulin was affected by the interaction of diet × day (P ≤ 0.05) because of the gradual decrease in OVE at 14 d (Table 2).
Blood Metabolites and Hormones during TZD Administration
In OVE as well as CON cows, TZD administration increased the concentrations of insulin, glucose, and BHBA (Table 3). The concentrations of insulin and BHBA were higher (P ≤ 0.05), and concentrations of NEFA and ADIPOQ lower in OVE compared with CON cows (Table 3). The TZD did not affect (P > 0.10) the serum concentrations of NEFA and ADIPOQ (Table 3). The ratio of glucose and NEFA to insulin decreased (P < 0.05) in OVE compared with CON, or in response to TZD (Table 2).
TZD Concentration in Blood
The main effects of diet, time, and interaction on serum TZD are reported in Fig 1. An interaction (diet × day, P ≤ 0.05) was observed because of a lower blood TZD concentration in OVE at 18 d post-injection and for CON at 20 d post-injection. Those responses were followed by lower blood TZD concentration in both treatment groups from d 20 to 35. The concentration of TZD in blood was lower in OVE compared with CON over time. There was no overall effect (P > 0.05) of diet on TZD concentration in blood (Fig 1).
Gene Expression
Subcutaneous Adipose Tissue. The main effects of diet, TZD, and interactions for the genes in SAT are reported in Fig 2 and Table 4. The mRNA expression of SLC2A4, ADIPOQ FASN, DGAT2, INSIG1, SUMO1, and UBC9 was overall greater (diet, P < 0.05) in OVE compared with CON cows (Fig 2; Table 4). The mRNA expression of insulin signaling (INSR, IRS1, and SLC2A4), lipogenic enzymes/inducers (SCD, INSIG1, and DGAT2), post-translational modifiers (SUMO1, and UBC9), and ADIPOQ increased (P ≤ 0.05) at 1 weeks after TZD administration, but subsequently decreased (P ≤ 0.05) at 2 weeks post-TZD administration. Their expression increased again to values observed after 1 week of TZD at the end of the washout period (Fig 2; Table 4).
Despite the up-regulation of insulin sensitivity-related genes, the mRNA expression of the adipogenic gene PPARG and its lipogenic target FASN, as well as the insulin responsive transcription regulator SREBF1, decreased at 1 week after TZD administration, and subsequently increased (P ≤ 0.05) at 2 weeks post-TZD administration. Opposite to the response detected for insulin signaling and post-translational modifiers, the expression of PPARG, FASN, and SREBF1 decreased again at the end of the washout period (Fig 2; Table 4).
Skeletal Muscle Tissue. The main effects of diet, TZD, and interactions for the genes in skeletal muscle tissue before and during TZD administration are reported in Fig 3 and Table 4. The OVE cows had lower overall mRNA expression of PDK4 (Table 4), and its expression decreased at 1 week after TZD administration. Expression of PDK4 subsequently returned to the same values detected on d 14 at the end of the TZD treatment. The mRNA expression of insulin signaling (SLC2A4) and fatty acid oxidation genes (PPARA and ACADVL) was greater (P ≤ 0.05) at 2 weeks after diet initiation and 1 week after TZD administration compared with 2 weeks after TZD administration (Fig 3; Table 4). The OVE cows had a lower (P < 0.05) overall mRNA expression of CPT1A and ACOX1 compared with CON cows. The main effect of diet and interaction were due to the lower mRNA expression of ACOX1 in OVE cows on d 21 (Fig 3). Regardless of diet, TZD administration resulted in modest up-regulation (P ≤ 0.05) of CPT1A at 2 weeks post-TZD (Table 4). Compared with CON cows, OVE cows had greater (P < 0.05) mRNA expression of the glyceroneogenesis-related genes PCK1 and PC. An interaction (diet × day, P ≤ 0.05) for the mRNA expression of PCK1 and PC was observed because of a decrease in mRNA expression of both genes in OVE cows at 28 d, 2 weeks after the TZD administration (Fig 3). No effect (P > 0.10) of diet, TZD or the interaction was observed for PPARD and DGAT1.
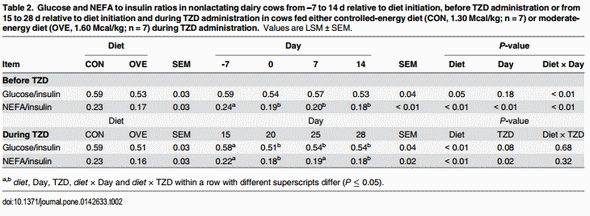
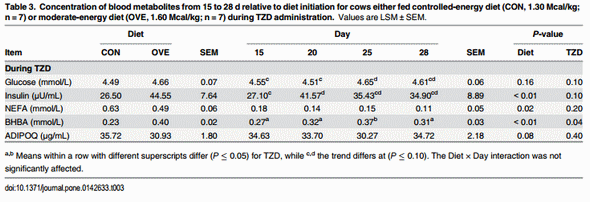
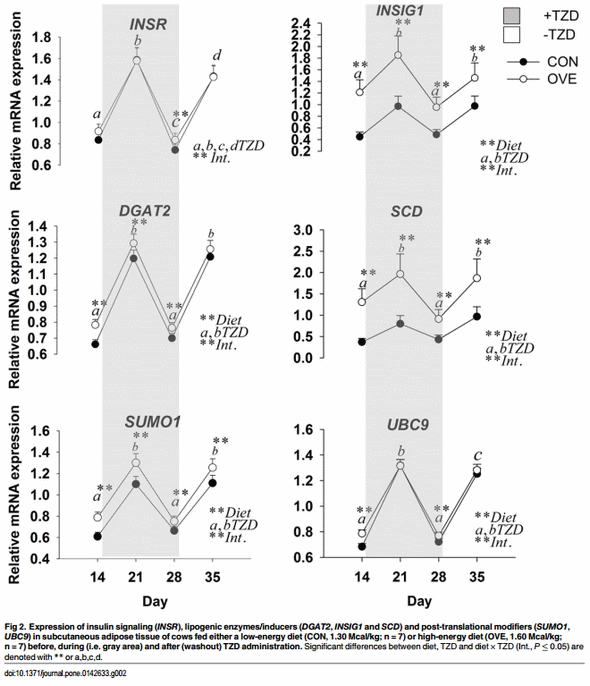
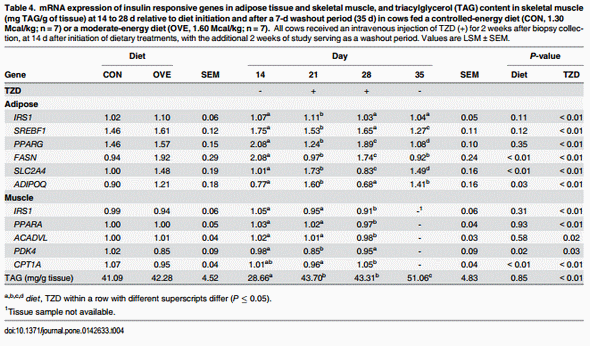
Skeletal Muscle Tissue Triacylglycerol Concentrations
The main effect of TZD was due to the greater triacylglycerol (TAG) synthesis during the TZD administration period and washout period at d 21, 28 and 35 (P ≤ 0.08; Table 4). No effect (P > 0.10) of diet or the interaction was observed for TAG in skeletal muscle.
Discussion
Studies using euglycemic clamps [17] and glucose tolerance tests (GTT) [5] demonstrated that late-pregnancy in sheep and cows is characterized by altered insulin sensitivity. Decreased insulin sensitivity prepartum and early postpartum contributes to the typical increase in circulating NEFA and it has been speculated that such response is one of the causes for the decrease in voluntary DMI after calving [18, 19]. Previous data from our group provided evidence that overfeeding a higher-energy diet during the last ~20 to 30 d prepartum did not impair SAT insulin signal transduction but resulted in greater NEFA postpartum [20]. The higher NEFA and lower DMI relationship postpartum is associated with several periparturient health disorders, e.g. displaced abomasum, ketosis, fatty liver, retained placenta, metritis, and mastitis. The administration of TZD (4 mg of TZD/kg of BW) during the late prepartum period led to greater concentration of blood insulin the first week postpartum [6], which likely accounted for the lower NEFA, i.e. the drug probably induced greater insulin sensitivity in AT [7].
The nuclear receptor PPARG is central for the maintenance of adipogenesis, hence, targeting this receptor during the late prepartum period has the potential to improve metabolic health and DMI of periparturient dairy cows [2]. In order to study in more detail the mechanistic regulation of insulin sensitivity at the molecular and whole-animal level we utilized non-lactating and non-pregnant dairy cows fed to meet energy requirements or allowed to consume energy ad-libitum in comparison with cows limited to near their energy requirements. The experimental period used was approximately the length of a typical nonlactating period with the caveat that the responses of non-pregnant cows to higher dietary energy might differ from pregnant cows due to nutrient requirements and endocrine environment [21].
Energy Intake Response
The greater NEL intake throughout the study in cows fed the OVE diet reinforces other observations that dairy cows do not control energy intake or that the set-points above which voluntary energy intake reaches a limit were not attained. Consequently, excess dietary energy intake resulted in surplus that accumulated in fat depots as we reported recently [21]. Two comprehensive reviews underscored that regulation of DMI in dairy cow occurs through a variety of mechanisms including hepatic oxidation of fuels as well as signals reaching brain centers regulating satiety [18, 22]. The results of a study conducted by Smith et al. [4] revealed that administration of 4 mg TZD/kg of BW once daily from 21 d prepartum to parturition increased DMI only during d -7 through +7. The fact that NEL intake in the current study remained the same during TZD administration and gradually increased in OVE cows during the washout period partly agrees with results from Smith et al. [4]. At a mechanistic level, an effect of PPARG agonists on brain centers regulating satiety cannot be discounted because PPARG activation by TZD increased feed intake in rodents without changing hypothalamic neuropeptide Y gene expression [23, 24]. The effect of TZD on bovine hypothalamic genes remains to be determined.
The lower NEFA and higher insulin concentrations over time before TZD administration in OVE cows indicated that energy balance was positive and insulin sensitivity was normal. These results agree with data from late-pregnant cows overfed energy [20]. According to Allen et al. [22] insulin might affect voluntary DMI indirectly through increasing hepatic oxidation of primary and non-primary gluconeogenic precursors (e.g., propionate and butyrate). Such response would occur by increasing the clearance of fuels from the blood, and by decreasing AT lipolysis and supply of NEFA to the liver. In our study, greater insulin in OVE cows did not seem to suppress the NEL intake. Therefore, our data highlight that voluntary feed intake is regulated by multiple factors with alternative mechanisms of action [22].
The unchanged blood concentration of glucose along with greater concentration of insulin before TZD administration in OVE cows was expected. There were positive correlations between NEFA and glucose, and between insulin and BHBA (Table 5). A GTT indicated that in dairy cows fed high- and low-energy diets glucose concentration does not differ greatly [5]. Therefore, the effect of diet on blood glucose clearance and metabolism in the present study was minimal despite elevated concentration of insulin in the OVE cows. The observed responses in glucose and BHBA during TZD agree with previous results [6, 25]. Positive correlations were detected between glucose and insulin, BHBA and insulin before and during TZD administration, and between BHBA and glucose. In contrast, insulin had a positive and NEFA a negative correlation with BHBA (Table 5). The positive correlation between the BHBA and insulin before and during TZD administration likely was due to greater rumen butyrate production as a consequence of greater DMI in OVE cows. The results of previous studies indicated that in non-ruminants, TZD increases glucose utilization in AT, muscle and liver, while it decreases glucose production [26, 27].
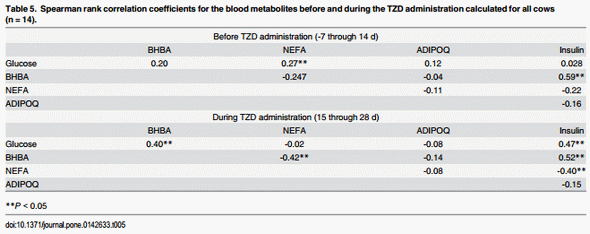
Our results of serum ADIPOQ are in accordance with data from mice in which lower energy intake led to lower white AT mass but increased blood levels of ADIPOQ [28]. While seemingly a paradox, the greater mRNA abundance of ADIPOQ in SAT concomitant with lower serum concentration of ADIPOQ in OVE cows might be expected because in obese subjects during and after diet-induced weight loss there was a dissociation between AT mRNA expression and serum levels of ADIPOQ [29]. The lack of association between AT mRNA expression and serum concentration of ADIPOQ, the mechanistic role for ADIPOQ in regulating DMI, and the link between insulin sensitivity and regulation of ADIPOQ in bovine deserve further study.
Circulating ADIPOQ concentration is inversely correlated with body fat content in nonruminants [30], and our data of increased BW in OVE compared with CON cows supports this relationship. In mice, ADIPOQ has no effect on insulin secretion by islets, but can enhance glucose-stimulated insulin secretion in islets from mice with diet-induced obesity [31]. Our results do not suggest any change in the dynamics of blood glucose concentration before TZD administration. However, at 7 to 14 d (Fig 1) there was a trend for a negative correlation between insulin concentration and ADIPOQ concentration in both treatment groups; this is in accordance with a previous study in which insulin inhibited the expression of ADIPOQ in cultured bovine adipocytes [32].
In non-ruminants, the preponderance of data indicate that the TZD class of PPARG agonists are potent inducers of ADIPOQ expression [33]. In the current study, the expression of ADIPOQ and insulin-related genes after 7 d of TZD injection responded in a similar fashion as the serum concentration of ADIPOQ. Furthermore, their expression along with serum ADIPOQ decreased after 14 d of TZD injection. The ADIPOQ mRNA and serum response to TZD administration is consistent with the presumed higher insulin sensitivity. Differences in ADIPOQ mRNA and serum concentration in our data are in agreement with recent findings in which serum ADIPOQ was positively correlated with ADIPOQ protein concentrations [34].
TZD, PPAR, and Insulin Sensitivity
A recent comprehensive review on PPAR in ruminants highlighted that PPARA and PPARD are involved in the regulation β-oxidation of long-chain fatty acids in liver and skeletal muscle, and PPARG in controlling adipogenesis and insulin sensitivity in AT [2]. The expression of both PPARA and PPARD increased in liver of ketotic cows, a response ascribed in part to the greater influx of NEFA from lipolysis of AT [35]. Although PPARD expression in muscle tissue was undetectable in the present study, the decrease in mRNA expression of PPARA along with several targets decreased 2 weeks post-TZD administration, which suggests a decrease in fatty acid oxidation and a potential increase in TAG accumulation [36].
The increase in insulin sensitivity in AT via activation of PPARG due to exogenous TZD in non-ruminants is well characterized [3]. However, in bovine, only a few studies with conflicting outcomes support the effect of TZD on PPARG expression [5, 37]. Although only a numerically-greater effect of diet on PPARG protein expression was detected in OVE cows, the mRNA expression of PPARG was altered during the TZD administration in accordance with Schoenberg et al. [7]. It is possible that TZD restores the insulin sensitivity in bovine through PPARG via alternate mechanisms, e.g. switching oxidative metabolism to fat synthesis in addition to the ultimate sequestration of fatty acids into TAG. Furthermore, the degree of insulin responsiveness in the skeletal muscle might be influenced indirectly by simultaneous effects on PPARG activation in other tissues such as AT [38].
TZD, Insulin, and Lipogenic Genes
In humans, the half-life of an insulin sensitizer such as rosiglitazone was 3 to 7 h after i.v. administration [39]. Our gene expression data highlighted that in both dietary groups the daily injection of TZD had an insulin-sensitizing effect in SAT at 21 d relative to diet initiation, but disappeared by d 28 when the last dose of TZD was injected. The results of a study in rodents indicated that the lack of in vitro response to TZD after 5 h might have been due to a slow rate of PPARG activation. The slower in vivo response in skeletal muscle insulin sensitivity could be due to an indirect effect of PPARG activation in AT. We speculate that the coordination between AT and skeletal muscle exists as an indirect mechanism, whereby the regulation of the insulin-responsive genes in skeletal muscle is influenced as a consequence of PPARG activation in another tissue such as AT. Greater TZD concentration at about 21 d relative to diet initiation in OVE cows might have increased the mRNA abundance of insulin sensitivity-related genes in AT, while greater blood TZD clearance at 28 d in OVE cows might have suppressed the mRNA expression of insulin sensitivity-related genes. The greater TZD response in OVE compared with CON cows might be related to the quantity of deposited AT in OVE cows, partly because the main target organ for TZD in bovine appears to be AT, which contains a greater number of PPARG receptors [2]. Perhaps the clearest evidence of an insulin-sensitizing effect of TZD in SAT was the differential change in mRNA expression of SREBF1 in parallel with INSR, INSIG1, SCD, SLC2A4, and IRS1 at 21 and 28 d. In obese mice, the AT is able to store additional TAG through the adaptive changes of INSIG1, SREBF1, and SCD [40]. The decreased mRNA expression of these genes at 4 weeks after diet initiation and during TZD administration might be due to selective modulation of PPARG occupancy at target gene promoters in AT. Increased mRNA expression of SREBF1 is potentially indicative of enhanced lipogenesis in TZD-injected cows, supporting a role of this transcription regulator in bovine SAT as in rodents [41].
Among the genes measured in the present study, SCD has been associated with diet-induced changes in insulin sensitivity in rodents. For instance, SCD1 knock-out mice fed a high-carbohydrate or high-fat diet remained lean and insulin sensitive, and had reduced AT lipolytic activity and greater hepatic β-oxidation [42]. Work in bovine revealed that SCD expression in SAT increased after beef steers were weaned to a higher-starch diet [43], confirming greater adipogenesis during growth of the animals.
In our study, the increased expression of SCD on d 21 after TZD might be linked with glucose metabolism in AT and improved glucose disposal induced by the PPARG agonist. In fact, in the overall context of TAG synthesis, the greater expression of SCD and DGAT2 on d 21 suggests an acute enhancement of insulin sensitivity by the sustained increase in circulating insulin. In male rats, rosiglitazone increased the mRNA expression of DGAT2. The return to basal expression for both genes on d 28 might have occurred because of greater blood TZD clearance and/or lack of the free receptors due to daily administration of TZD on a daily dosage. Despite the lack of an interaction effect for mRNA expression of PPARG, the upregulation of PPARG targets such as FASN and DGAT2 prior to TZD was indicative of greater lipogenesis in OVE cows. The above observations, coupled with the differential expression of PPARG target genes, underscore that PPARG is a major player in SAT.
Glyceroneogenesis and Insulin Sensitivity in Skeletal Muscle
Glyceroneogenesis is an important pathway in the recycling of intracellular NEFA towards TAG synthesis. The study of Tordjman et al. [44] highlighted that exogenous TZD improves insulin sensitivity partly through induction of glyceroneogenesis, leading to a decrease in NEFA release from fat cells. The TAG/fatty acid cycle includes local intracellular cycling within the AT and extracellular or systemic recycling, as well as formation of the TAG in liver, AT, and muscle. In our study, the significant increase in TAG during the TZD injection and washout period supports the potential sensitizing effect of TZD on the skeletal muscle, hence, the TZD might have led to greater glucose disposal into TAG. The synthesis of TAG in skeletal muscle of non-ruminants relies heavily on the expression and activity of PCK1 [45]. Regardless of TZD, the greater expression of PC and PCK1 coupled with lower expression of PDK4 (which is upregulated during negative energy balance; Bionaz et al. [2]) in OVE compared with CON cows might be linked to generation of glycerol-3-phosphate for TAG synthesis through glyceroneogenesis (rather than glycolysis) utilizing a non-glucose precursor (e.g. lactate). This idea is supported by the greater mRNA expression of SLC2A4 in cows fed CON, suggesting that the skeletal muscle of these cows transported more glucose not only for glycerol-3-phosphate but also to produce ATP through the TCA cycle. These adaptations also are supported by the greater NEFA concentration in CON cows, which might have provided fatty acids as sources of energy in liver and skeletal muscle.
In ruminants, the greater NEFA surge into plasma after parturition was associated with greater expression of PPARA target genes such as CPT1A, ACOX1 and ACADVL [46]. Positive correlations between plasma BHBA concentration and CPT1A and ACADVL in liver of dairy cows were reported [46]. However, in diet-induced obese mice the inhibition of CPT1 activity alleviates insulin resistance [47]. Therefore, in our study the lower mRNA expression of CPT1A and PDK4 in the skeletal muscle of OVE cows offers additional support for greater insulin sensitivity in these cows.
Post Translational Modifiers: Novel Link with Adipogenesis
Another mechanism of control over PPARG activity involves its SUMOylation, which refers to the reversible covalent attachment of small ubiquitin-like modifier (SUMO) peptides (SUMO 1, -2, -3 in mammals). The process involves an activating enzyme (SAE1/SAE2), a conjugating enzyme (UBC9), and an E3 ligase that typically leads to repression of transcription factors [48]. A comprehensive recent review in non-ruminant species underscored the multiple cross-talks between mRNA biogenesis and SUMO proteins in triggering the adipogenesis-responsive factors such as PPAR and SREBP [49]. The opposite expression of SUMO1 and UBC9 compared with PPARG and SREBF1 in the present study seems to agree with non-ruminant data. We speculate that the upregulation of post-translational modifiers during the first 7 d of TZD injection was a response to balance the energy expenditure and fine-tune the metabolic master regulators in an autocrine, endocrine, and paracrine fashion in SAT [48]. The moderately greater expression of mRNA of SUMO1 and UBC9 on d 21 and 28 in OVE compared with CON cows might be due to the role of post-translational modifiers to “brake” the processes of adipogenesis and lipogenesis initiated and sustained by PPARG and SREBF1. Therefore, the observed effect of TZD on SUMO1 and UBC9 would serve as a negative feedback to coordinate adaptive mechanisms of PPARG and SREBF1 to TZD.
Conclusions
The ad-libitum access to a moderate-energy diet markedly increased the energy balance and up-regulated mRNA expression of lipogenic and insulin-induced glucose transport related genes in AT, suggesting that the additional energy did not cause satiety or alter insulin sensitivity. Level of energy intake caused differential TZD clearance rates during its administration, but the TZD was effective in up-regulating PPARG and several target genes in AT during the first 7 d regardless of diet. The fact that these genes are PPARG-targets in non-ruminants suggests that, similar to non-ruminant species, the bovine PPARG receptor in dairy cow also is TZD-responsive. Hence, as suggested in previous studies, targeting PPARG during late-pregnancy through early lactation when insulin concentration and sensitivity decreases might be a practical tool to prevent excessive losses in body condition.
Supporting Information
S1 File. Management and sampling. Tail-head adipose tissue biopsy. Hind-leg muscle biopsy. The TZD preparation. RNA extraction, quality assessment, and cDNA synthesis. Primer design and evaluation. Quantitative PCR (qPCR). Relative mRNA abundance of genes within adipose tissue and muscle tissues. Western blot analysis of PPARG within adipose tissue. Table A. GenBank accession number, sequence and amplicon size of primers used to analyze gene expression by quantitative PCR. Table B. Gene symbol, gene name, and description of the main biological function and biological processes of the targets analyzed in subcutaneous adipose tissue and muscle. Table C. Sequencing results obtained from PCR products. Table D. qPCR performance among the genes measured in adipose tissue and muscle. Figure A. Summary of potential regulatory network of genes involved in insulin response in subcutaneous adipose tissue. Figure B. Summary of Diet × Time effects on regulatory network of genes involved in insulin response in subcutaneous adipose tissue. Figure C. Summary of potential regulatory network of genes involved in insulin response in muscle tissue. Figure D. Summary of Diet × Time effects on regulatory network of genes involved in insulin response in muscle tissue. (DOCX)
S2 File. Western blot data. (XLSX)
Author Contributions
Conceived and designed the experiments: JJL AH JKD. Performed the experiments: AH MRT FTdR ZI OM JK. Analyzed the data: AH. Contributed reagents/materials/analysis tools: OM HS JK ET. Wrote the paper: AH JJL.
This article was originally published in PLoS ONE 10(11): e0142633. doi:10.1371/journal.pone.0142633. This is an Open Access article distributed under the terms of the Creative Commons Attribution License.
References
1. Desvergne B, Wahli W. Peroxisome proliferator-activated receptors: nuclear control of metabolism. Endocr Rev. 1999; 20:649–88. PMID: 10529898
2. Bionaz M, Chen S, Khan MJ, Loor JJ. Functional Role of PPARs in Ruminants: Potential Targets for Fine-Tuning Metabolism during Growth and Lactation. PPAR research. 2013; 2013:684159. doi: 10. 1155/2013/684159 PMID: 23737762
3. Ahmadian M, Suh JM, Hah N, Liddle C, Atkins AR, Downes M, et al. PPARgamma signaling and metabolism: the good, the bad and the future. Nat Med. 2013; 19:557–66. doi: 10.1038/nm.3159 PMID: 23652116
4. Smith KL, Butler WR, Overton TR. Effects of prepartum 2,4-thiazolidinedione on metabolism and performance in transition dairy cows. J Dairy Sci. 2009; 92:3623–33. doi: 10.3168/jds.2008-1508 PMID: 19620643
5. Schoenberg KM, Overton TR. Effects of plane of nutrition and 2,4-thiazolidinedione on insulin responses and adipose tissue gene expression in dairy cattle during late gestation. J Dairy Sci. 2011; 94:6021–6035. doi: 10.3168/jds.2011-4533 PMID: 22118090
6. Smith KL, Stebulis SE, Waldron MR, Overton TR. Prepartum 2,4-thiazolidinedione alters metabolic dynamics and dry matter intake of dairy cows. J Dairy Sci. 2007; 90:3660–70. PMID: 17638977
7. Schoenberg KM, Perfield KL, Farney JK, Bradford BJ, Boisclair YR, Overton TR. Effects of preparum 2,4-thiazolidinedione on insulin sensitivity, plasma concentrations of tumor necrosis factor-alpha and leptin, and adipose tissue gene expression. J Dairy Sci. 2011; 94:5523–32. doi: 10.3168/jds.2011-4501 PMID: 22032375
8. AVANDIA1 (rosiglitazone maleate) [Internet]. Food and Drug Administration (FDA). 2007 [cited 2014].
9. Bionaz M, Trevisi E, Calamari L, Librandi F, Ferrari A, Bertoni G. Plasma paraoxonase, health, inflammatory conditions, and liver function in transition dairy cows. J Dairy Sci. 2007; 90:1740–50. PMID: 17369214
10. Mielenz M, Mielenz B, Singh SP, Kopp C, Heinz J, Haussler S, et al. Development, validation, and pilot application of a semiquantitative Western blot analysis and an ELISA for bovine adiponectin. Domest Anim Endocrinol. 2013; 44:121–30. doi: 10.1016/j.domaniend.2012.10.004 PMID: 23291015
11. Sosa C, Abecia JA, Carriquiry M, Forcada F, Martin GB, Palacin I, et al. Early pregnancy alters the metabolic responses to restricted nutrition in sheep. Domest Anim Endocrinol. 2009; 36:13–23. doi: 10. 1016/j.domaniend.2008.08.003 PMID: 18838244
12. Gassmann M, Grenacher B, Rohde B, Vogel J. Quantifying Western blots: pitfalls of densitometry. Electrophoresis. 2009; 30:1845–55. doi: 10.1002/elps.200800720 PMID: 19517440
13. Tan HY, Ng TW. Accurate step wedge calibration for densitometry of electrophoresis gels. Optics Communications. 2008; 281:3013–7.
14. Abramoff MD, Magalhaes PJ, Ram SJ. Image Processing with ImageJ. Biophotonics International. 2004; 11:36–42.
15. Ravikanth C, Kumar A, Kiran U, Prashanth S, Madhu B, Reddy Y. Sensitive and Rapid HPLC method for the determination of pioglitazone in rat serum. Int. J. Pharm. Sci. Drug Res. 2011; 3:38–41.
16. Giaginis C, Theocharis S, Tsantili-Kakoulidou A. Investigation of the lipophilic behavior of some thiazolidinediones. Relationships with PPAR-γ activity. J Chromatogr B Analyt Technol Biomed Life Sci. 2007; B. 857:181–7. PMID: 17660053
17. Petterson JA, Dunshea FR, Ehrhardt RA, Bell AW. Pregnancy and undernutrition alter glucose metabolic responses to insulin in sheep. J Nutr. 1993; 123:1286–95. PMID: 8320567
18. Allen MS, Bradford BJ, Harvatine KJ. The cow as a model to study food intake regulation. Annu Rev Nutr. 2005; 25:523–47. PMID: 16011477
19. Khan MJ, Jacometo CB, Graugnard DE, Correa MN, Schmitt E, Cardoso F, et al. Overfeeding Dairy Cattle During Late-Pregnancy Alters Hepatic PPARalpha-Regulated Pathways Including Hepatokines: Impact on Metabolism and Peripheral Insulin Sensitivity. Gene Regul Syst Bio. 2014; 8:97–111. doi: 10.4137/GRSB.S14116 PMID: 24737933
20. Ji P, Osorio JS, Drackley JK, Loor JJ. Overfeeding a moderate energy diet prepartum does not impair bovine subcutaneous adipose tissue insulin signal transduction and induces marked changes in peripartal gene network expression. J Dairy Sci. 2012; 95:4333–51. doi: 10.3168/jds.2011-5079 PMID: 22818447
21. Drackley JK, Wallace RL, Graugnard D, Vasquez J, Richards BF, Loor JJ. Visceral adipose tissue mass in nonlactating dairy cows fed diets differing in energy density(1). J Dairy Sci. 2014; 97:3420–30. doi: 10.3168/jds.2014-8014 PMID: 24704224
22. Allen MS, Bradford BJ, Oba M. Board Invited Review: The hepatic oxidation theory of the control of feed intake and its application to ruminants. J Anim Sci. 2009; 87:3317–3334. doi: 10.2527/jas.2009- 1779 PMID: 19648500
23. Larsen PJ, Jensen PB, Sorensen RV, Larsen LK, Vrang N, Wulff EM, et al. Differential influences of peroxisome proliferator-activated receptors gamma and -alpha on food intake and energy homeostasis. Diabetes. 2003; 52:2249–59. PMID: 12941763
24. Wolden-Hanson T, Marck BT, Matsumoto AM. Troglitazone treatment of aging Brown Norway rats improves food intake and weight gain after fasting without increasing hypothalamic NPY gene expression. Exp Gerontol. 2002; 37:679–91. PMID: 11909685
25. Schoenberg KM, Ehrhardt RM, Overton TR. Effects of plane of nutrition and feed deprivation on insulin responses in dairy cattle during late gestation. J Dairy Sci. 2012; 95:670–82. doi: 10.3168/jds.2011- 4529 PMID: 22281332
26. Iwamoto Y, Kosaka K, Kuzuya T, Akanuma Y, Shigeta Y, Kaneko T. Effects of troglitazone: a new hypoglycemic agent in patients with NIDDM poorly controlled by diet therapy. Diabetes Care. 1996; 19:151– 6. PMID: 8718436
27. Nolan JJ, Ludvik B, Beerdsen P, Joyce M, Olefsky J. Improvement in glucose tolerance and insulin resistance in obese subjects treated with troglitazone. N Engl J Med. 1994; 331:1188–1193. PMID: 7935656
28. Qiao L, Lee B, Kinney B, Yoo HS, Shao J. Energy intake and adiponectin gene expression. Am J Physiol Endocrinol Metab. 2011; 300:E809–816. doi: 10.1152/ajpendo.00004.2011 PMID: 21325106
29. Behre CJ, Gummesson A, Jernas M, Lystig TC, Fagerberg B, Carlsson B, et al. Dissociation between adipose tissue expression and serum levels of adiponectin during and after diet-induced weight loss in obese subjects with and without the metabolic syndrome. Metabolism. 2007; 56:1022–1028. PMID: 17618945
30. Kadowaki T, Yamauchi T, Kubota N, Hara K, Ueki K, Tobe K. Adiponectin and adiponectin receptors in insulin resistance, diabetes, and the metabolic syndrome. J Clin Invest. 2006; 116:1784–92. PMID: 16823476
31. Winzell MS, Nogueiras R, Dieguez C, Ahren B. Dual action of adiponectin on insulin secretion in insulin-resistant mice. Biochem Biophys Res Commun. 2004; 321:154–60. PMID: 15358228
32. Sun YG, Zan LS, Wang HB, Guo HF, Yang DP, Zhao XL, et al. Insulin Inhibits the Expression of Adiponectin and AdipoR2 mRNA in Cultured Bovine Adipocytes. Seoul, COREE, REPUBLIQUE DE: Asianaustralas. J. Anim. Sci.; 2009. 8 p.
33. Dadson K, Liu Y, Sweeney G. Adiponectin action: a combination of endocrine and autocrine/paracrine effects. Front Endocrinol (Lausanne). 2011; 2:62.
34. Singh SP, Haussler S, Heinz JF, Akter SH, Saremi B, Muller U, et al. Lactation driven dynamics of adiponectin supply from different fat depots to circulation in cows. Domest Anim Endocrinol. 2014; 47:35– 46. doi: 10.1016/j.domaniend.2013.12.001 PMID: 24462180
35. Loor JJ, Everts RE, Bionaz M, Dann HM, Morin DE, Oliveira R, et al. Nutrition-induced ketosis alters metabolic and signaling gene networks in liver of periparturient dairy cows. Physiol Genomics. 2007; 32:105–116. PMID: 17925483
36. Burri L, Thoresen GH, Berge RK. The Role of PPARalpha Activation in Liver and Muscle. PPAR research. 2010; 2010.
37. Arevalo-Turrubiarte M, Gonzalez-Davalos L, Yabuta A, Garza JD, Davalos JL, Mora O, et al. Effect of 2,4-thiazolidinedione on limousin cattle growth and on muscle and adipose tissue metabolism. PPAR research. 2012; 2012:891841. doi: 10.1155/2012/891841 PMID: 23304114
38. Zierath JR, Ryder JW, Doebber T, Woods J, Wu M, Ventre J, et al. Role of skeletal muscle in thiazolidinedione insulin sensitizer (PPARgamma agonist) action. Endocrinology. 1998; 139:5034–41. PMID: 9832442
39. Cox PJ, Ryan DA, Hollis FJ, Harris AM, Miller AK, Vousden M, et al. Absorption, disposition, and metabolism of rosiglitazone, a potent thiazolidinedione insulin sensitizer, in humans. Drug Metab Dispos. 2000; 28:772–80. PMID: 10859151
40. Carobbio S, Hagen RM, Lelliott CJ, Slawik M, Medina-Gomez G, Tan CY, et al. Adaptive changes of the Insig1/SREBP1/SCD1 set point help adipose tissue to cope with increased storage demands of obesity. Diabetes. 2013; 62:3697–3708. doi: 10.2337/db12-1748 PMID: 23919961
41. Sekiya M, Yahagi N, Matsuzaka T, Takeuchi Y, Nakagawa Y, Takahashi H, et al. SREBP-1-independent regulation of lipogenic gene expression in adipocytes. J Lipid Res. 2007; 48:1581–91. PMID: 17456898
42. Rahman SM, Dobrzyn A, Dobrzyn P, Lee SH, Miyazaki M, Ntambi JM. Stearoyl-CoA desaturase 1 deficiency elevates insulin-signaling components and down-regulates protein-tyrosine phosphatase 1B in muscle. Proc Natl Acad Sci USA. 2003; 100:11110–5. PMID: 12960377
43. Martin GS, Lunt DK, Britain KG, Smith SB. Postnatal development of stearoyl coenzyme A desaturase gene expression and adiposity in bovine subcutaneous adipose tissue. J Anim Sci. 1999; 77:630–6. PMID: 10229358
44. Tordjman J, Chauvet G, Quette J, Beale EG, Forest C, Antoine B. Thiazolidinediones block fatty acid release by inducing glyceroneogenesis in fat cells. J Biol Chem. 2003; 278:18785–90. PMID: 12644461
45. Hanson RW, Hakimi P. Born to run; the story of the PEPCK-Cmus mouse. Biochimie. 2008; 90:838– 42. doi: 10.1016/j.biochi.2008.03.009 PMID: 18394430
46. Loor JJ, Dann HM, Everts RE, Oliveira R, Green CA, Guretzky NA, et al. Temporal gene expression profiling of liver from periparturient dairy cows reveals complex adaptive mechanisms in hepatic function. Physiol Genomics. 2005; 23:217–226. PMID: 16091418
47. Keung W, Ussher JR, Jaswal JS, Raubenheimer M, Lam VH, Wagg CS, et al. Inhibition of carnitine palmitoyltransferase-1 activity alleviates insulin resistance in diet-induced obese mice. Diabetes. 2013; 62:711–20. doi: 10.2337/db12-0259 PMID: 23139350
48. van Beekum O, Fleskens V, Kalkhoven E. Posttranslational modifications of PPAR-gamma: fine-tuning the metabolic master regulator. Obesity (Silver Spring). 2009; 17:213–9.
49. Rouviere JO, Geoffroy MC, Palancade B. Multiple crosstalks between mRNA biogenesis and SUMO. Chromosoma. 2013; 122:387–99. doi: 10.1007/s00412-013-0408-y PMID: 23584125