1. Introduction
Mycotoxins are fungal-generated secondary metabolites that are ubiquitous and unavoidable contaminants of food and feed, and their consumption by humans and animals results in diseases and death. Several species of the genus Aspergillus, Penicillium, and Fusarium are capable of producing mycotoxins (Cole & Cox, 1981). For instance, Aspergillus flavus, A. parasiticus, A. nomius, and A. psudotamarii are responsible for the production of a series of highly toxic substances, the aflatoxins (Varga, Frisvad, & Samson, 2009; Ito, Peterson, Wicklow, & Goto, 2001).
Among the aflatoxins synthesized by these fungi, aflatoxin B1 (AFB1) is considered the most powerful hepatocarcinogenic, hepatotoxic, immunosuppressive, and mutagenic agent known (Busby & Wogan, 1984). Because of the adverse effects of AFB1 on human and animal health, secure, practical, inexpensive, and effective decontamination strategies are highly desirable. Several approaches have been proposed to mitigate the impact of aflatoxins, the aim of these methods is either to remove, to inactivate, to transform, or to degrade the toxin and can be classified into physical, chemical, and biological (Rustom, 1997).
Currently, the most widely used physical decontamination strategy is adsorption of aflatoxins onto different materials. Inorganic binders such as phyllosilicates (bentonites, montmorillonites, smectites, kaolinites, and illites) and tectosilicates (zeolites); organic binders (yeast cell wall, lactic acid bacteria, micronized fibers, and activated carbon); and polymers (cholestyramine, divinylbenzene-styrene, polyvinylpyrrolidone, chitosan, hydroxypropyl methylcellulose, sodium carboxymethylcellulose, and microcrystalline cellulose) have been reported to remove AFB1 (Solís-Cruz et al., 2017; Vila-Donat, Marín, Sanchis, & Ramos, 2018). However, the most practical limiting factor is the high cost of some of these binders. Another potential disadvantage of these materials is the possibility of adsorption of important micronutrients (vitamins and minerals), and the release of toxic components such as heavy metals or dioxins
Recently, sorption with plant-derived materials has emerged as a potential alternative over the abovementioned AFB1 binding agents. This technology is based on a set of chemical and/or physical mechanisms including: hydrogen bonding, electrostatic attraction, ion exchange, chelation, precipitation, and complexation (Ringot et al., 2007).
Several studies have been conducted to evaluate the AFB1-sorption capacity of different biomaterials such as grape pomaces (Avantaggiato, Greco, Damascelli, Solfrizzo, & Visconti, 2014; Greco, D’Ascanio, Santovito, Logrieco, & Avantaggiato, 2019), banana peel (Shar et al., 2016), Pyracantha leaves and berries (Ramales-Valderrama, Vázquez-Durán, & Méndez-Albores, 2016), aloe powder (Zavala-Franco et al., 2018), and lignins from grassy plants (Karmanov et al., 2020). These materials have been reported to be effective in removing aflatoxins determined by different in vitro methodologies or simulated gastrointestinal digestion procedures (using consecutive incubation at different pH values, temperature, and enzymatic activities). However, there is currently a lack of information regarding the use of lettuce (Lactuca sativa L.) and field horsetail (Equisetum arvense L.) as sorbent biomaterials for AFB1 removal.
Based on the hypothesis that the biosorbents prepared from lettuce and field horsetail would exhibit both chemical and physical mechanisms to adsorb AFB1, the present research aims to: (i) assess the AFB1- sorption potential of two novel biomaterials – at low inclusion levels – when using an in vitro model simulating the pH conditions in the gastrointestinal tract of poultry, and (ii) evaluate the nature of the interaction between the biosorbents and the AFB1 molecule using several characterization methodologies.
2. Experimental
2.1. Chemicals
All chemicals were of analytical-reagent grade and used as received without further purification. Aflatoxin B1 from Aspergillus flavus (CAS number: 1162-65-8), dimethyl sulfoxide (≥99.9% purity, CAS number 67-68-5), HPLC grade acetonitrile (CAS number 75-05-8), HPLC grade methanol (CAS number 67-56-1), ethanol 96% (CAS number 64-17-5), and sodium hypochlorite solution (CAS number 7681-52-9) were obtained from Merck KGaA (Darmstadt, Germany).
2.2. Safety precautions
Sodium hypochlorite solution (5% w/v, available chlorine) was utilized to decontaminate laboratory equipment, working areas, and laboratory wastes. All aflatoxin-contaminated glassware was immersed in the solution overnight and then exhaustively washed.
2.3. Plant material and preparation of biosorbents
Samples of lettuce (Lactuca sativa L.), field horsetail (Equisetum arvense L.) and Formosa firethorn [Pyracantha koidzumii (Hayata) Rehder] cultivated in the Botanic Garden of the Superior Studies Faculty at Cuautitlan (National Autonomous University of Mexico) were collected during the winter and summer period in 2019. The methodology for biosorbent preparation has been previously described in detail by Ramales-Valderrama et al. (2016).
Briefly, the collected samples were washed repeatedly with distilled water to remove foreign materials. After cleaning, chopped materials were separately dried in an oven (Binder model RE-115, Tuttlingen, Germany) at 50 °C until no variation in the sample weight was recorded. Afterward, dried biosorbents were milled in an electric plate-style mill type C-11-1 (Glen Mills Inc., Clifton, NJ, USA) and sieved. Particles with an average size of < 250 µm (60 mesh) were used for biosorption.
2.4. Aflatoxin B1 (AFB1) preparation
A primary stock solution of AFB1 (100 µg/mL) was prepared in dimethyl sulfoxide (DMSO). Sorption experiments were carried out at different pH values using the following buffers: pH 2 (0.1 M KCl/HCl), pH 5 (0.15 M C6H8O7/Na2HPO4-2H2O), and pH 7 (0.1 M Na2HPO4- 7H2O/NaH2PO4H2O). Buffer solutions containing AFB1 were prepared using the primary stock solution in DMSO to attain a final concentration of 190 ng AFB1/mL. This concentration was chosen to reflect possible field conditions, and to approximately represent the Mexican limit for total aflatoxins (AFB1 + AFB2 + AFG1 + AFG2) in feed. The maximum limit for poultry is 100 ng/g and for swine up to 200 ng/g (NOM-188- SSA1-2002).
2.5. Batch experiments for AFB1 removal
A standard biosorption methodology was utilized to evaluate the effectiveness of biosorbents in removing AFB1 from buffer solutions. Samples of 25 mg (0.5% w/v) or 5 mg (0.1% w/v) of each biosorbent (lettuce, field horsetail, and Formosa firethorn) were weighed and dispersed in 5 mL of the buffer solution (at each particular pH) containing the AFB1 (190 ng/mL). The flasks were incubated (Bellco Glass Inc. NJ, USA) at 40 °C for 2 h with rotary agitation (120 rpm). After AFB1 adsorption, samples were centrifuged at 6300 × g (centrifuge 5810 R, Eppendorf, Germany) during 10 min, and the supernatant filtered through PTFE membrane syringe filter (pore size 0.22 μm)) and subjected to UPLC-FLR analysis. The pH was determined using a pH combination glass electrode (Conductronic PC-45, Puebla, Mexico). All determinations were performed in quintuplicate. Control samples (without adsorbent) were used to verify the stability of the AFB1 molecule in the different pH media under incubated conditions (40 °C for 2 h).
2.6. Aflatoxin B1 (AFB1) quantification
The AFB1 concentration in the filtrate was estimated by Ultra Performance Liquid Chromatography with fluorescence detection (UPLCFLR) using a Waters ACQUITY H-Class System according to the methodology suggested by Jardon-Xicotencatl, Díaz-Torres, Marroquín-Cardona, Villarreal-Barajas, and M´endez-Albores (2015). Briefly, an aliquot of the filtrate (10 µL) was separated in an ACQUITY UPLC BEH C18 column (2.1 × 100 mm, 1.7 μm) under isocratic conditions. The mobile phase consisted of HPLC grade water:methanol:acetonitrile (64:18:18) pumped with a flow rate of 0.4 mL/min by a quaternary solvent manager (QSM). Detection was achieved by an UPLC-optimized fluorescence detector (Waters, Milford, MA, USA) set at 365 nm excitation/429 nm emission wavelengths, respectively. Retention time was 3.92 min for AFB1 and a total run time of 8.0 min allowed return to initials conditions (Fig. S1, Supplementary data). The limit of detection for AFB1 was 2 ng/ L. The mean recovery for this methodology was 92%, with a standard error of 1.2, and a coefficient variation value of 4.7%. The AFB1 concentration was calculated using a standard reference with a calibration curve.
2.7. Bioisorbents characterization
The Fourier transform infrared (FTIR) spectra were acquired in a Frontier SP8000 spectrophotometer (Perkin Elmer, Waltham, MA, USA) accessorized with an in-compartment diamond Attenuated Total Reflectance (ATR) accessory (DuraSamplIR II, Smiths Detection, Warrington, UK). All spectra were collected in the range 4000–500 cm−1 with a resolution of 4 cm−1. The point of zero charge (pHpzc) was determined following the recommendations of Zavala-Franco et al. (2018). The electrophoretic mobility measurements and conversion to Zeta potentials (ζ) were made using the ZetaSizer Pro (Malvern Instruments, Worcestershire, UK) following the recommendations of Ramales-Valderrama et al. (2016).
Photosynthetic pigments (chlorophylls) were determined by measuring the absorbance of biosorbent extracts in 96% ethanol, as described by Wintermans and De Mots (1965). UV–Vis spectral analysis was performed by using a Cary 8454 UV–Vis Diode Array System spectrophotometer (Agilent Technologies, Santa Clara, CA, USA). Chlorophyll a (Chl a), chlorophyll b (Chl b), and total chlorophyll (Chl a + b) concentrations were calculated using the following equations:
Chl a = 13.70(A665) − 5.76(A649)
Chl b = 25.80(A649) − 7.60(A665)
Chla + b = 6.10(A665) + 20.04(A649)
2.8. Experimental design and statistical analysis
The experiment was conducted as a completely randomized 3 × 3 × 2 factorial design; eighteen experimental conditions were carried out with five replicates. The first factor corresponds to the biosorbent type (lettuce, field horsetail, and Formosa firethorn), the second factor corresponds to the pH values (2, 5, and 7), and the third factor corresponds to the sorbent content (0.5% and 0.1% w/v). Data were assessed by twoway analysis of variance (ANOVA), and means comparisons were performed according to the Tukey’s multiple range test (p < 0.05) using the Statistical Analysis System (SAS, 1990). A significance value of α = 0.05 was used to distinguish significant differences.
3. Results and discussion
3.1. Adsorption experiments
The three biosorbents (lettuce, field horsetail, and Formosa firethorn) were evaluated in different buffer solutions that simulated the in vivo conditions of the poultry gastrointestinal tract (proventriculus, pH 2; crop, pH 5; and intestine, pH 7). Our research group has been previously shown that one of these biosorbents (Formosa firethorn) had higher adsorption capacity of AFB1 (up to 86%) using an in vitro assay (Ramales-Valderrama et al., 2016), and moderate AFB1 biosorption uptake (up to 46%) using an in vitro multicompartmental model simulating the dynamic conditions in the gastrointestinal tract of poultry (Zavala-Franco et al., 2018). Consequently, in this research, Formosa firethorn was used as reference material.
The obtained results for AFB1 adsorption capacities are presented in Fig. 1. In general, when the biosorbent dosage was increased (0.5% w/v), AFB1 was well adsorbed by the three tested materials (Fig. 1, profile a); however, the adsorption of AFB1 by field horsetail was significantly lower at pH 2 (38 ± 9%). This effect could be due to changes in the charge of the adsorbent induced by the pH of the buffer solutions. Formosa firethorn (the reference material) yielded AFB1 reductions of 87 ± 6% at pH 5, which is in close agreement with our previous research (Ramales-Valderrama et al., 2016). At pH 7, the three biosorbents showed AFB1 reductions of almost 100% (Fig. 1, profile a). In this context, it is well known that the increase in the amount of the biosorbent increases the number of adsorptive sites responsible for the AFB1 uptake.
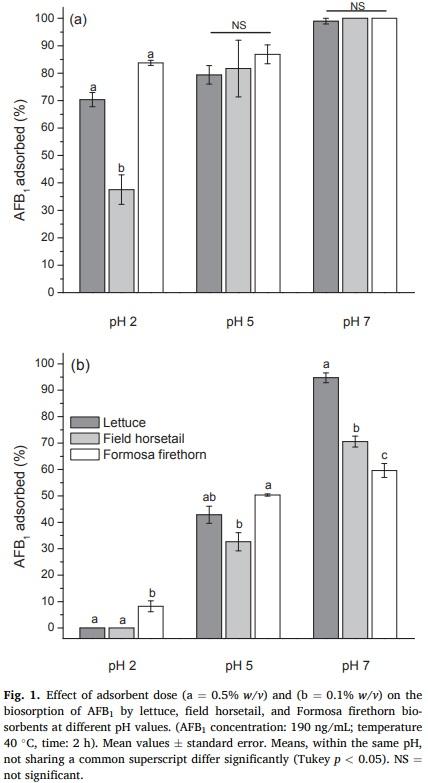
Furthermore, when the biosorbent dosage was decreased (0.1% w/v), minimal adsorption of AFB1 on the three tested biomaterials was observed at pH 2; however, significant AFB1 adsorption efficiencies were attained at pH 5. Lettuce, field horsetail, and Formosa firethorn adsorbed 43 ± 6%, 33 ± 5%, and 50 ± 1%, respectively. Nevertheless, at neutral pH, lettuce and field horsetail biosorbents showed the highest efficiencies against AFB1 removal, the biosorption percentages were 95 ± 3% and 71 ± 4%, respectively (Fig. 1, profile b). These results suggest that these two biosorbents show competitive adsorption capacities (at pH 7) for the removal of AFB1.
Table 1 presents the comparison of the AFB1 sorption capacity of the three tested materials with that of various plant-derived biosorbents reported in the literature (Avantaggiato, Greco, Damascelli, Solfrizzo, & Visconti, 2014; Fernandes, Calado, Guimaraes, ˜ Rodrigues, & Abrunhosa, 2019; Greco, D’Ascanio, Santovito, Logrieco, & Avantaggiato, 2019; Karmanov et al., 2020; Ramales-Valderrama et al., 2016; Shar et al., 2016; Zavala-Franco et al., 2018).
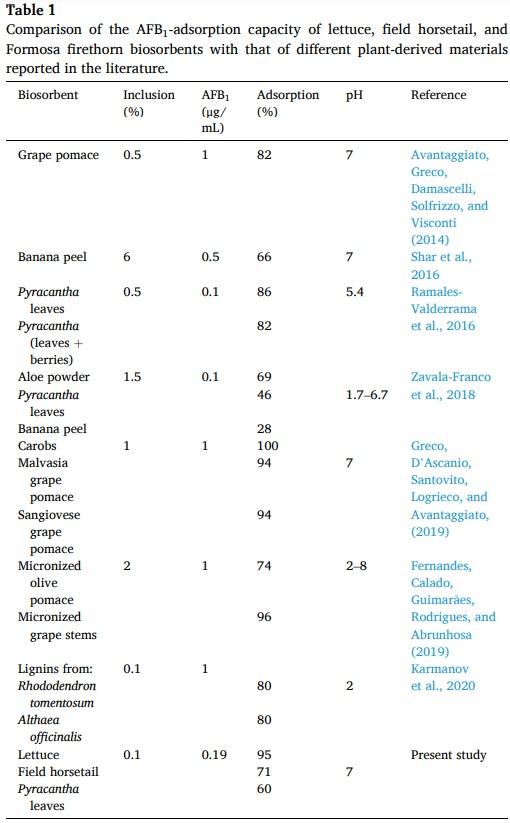
As can be seen, the biosorption capacity of lettuce (0.1% w/v biosorbent dose) is significantly higher than that of the majority of the biosorbents, with the exception of lignins. However, the methodology proposed in this work to prepare the biosorbent, offers the possibility to reduce the cost of production, considering that the management of agrowastes is an attractive option from the viewpoint of the waste recycle and minimization of contaminants such as AFB1. Therefore, lettuce biosorbent has significant potential for the removal of AFB1 in some gastrointestinal tract compartments of poultry such as the crop and intestine.
3.2. Fourier transform infrared spectroscopy with attenuated total reflection (FTIR-ATR) studies
The different extents of AFB1 adsorption onto the three tested materials suggested different sorption mechanisms according to their diverse composition. Therefore, biosorbents were further characterized to obtain information about the nature of the interaction between the functional groups present in the biomaterials and the AFB1 molecule. The FTIR-ATR spectra of unloaded and AFB1-loaded biosorbents were collected in the spectral range 4000–500 cm−1. A representative FTIR spectra comparison is showed in Fig. S2 (Supplementary data). Additionally, the primary active FTIR vibrations and their functional group or commonly assigned compound are shown in Table S1 (Supplementary data).
In general, biosorbents exhibited higher quantities of functional groups associated with the hydroxyl (3674–3282 cm−1), carboxyl and carboxylate (1733–1608 cm−1), amide (1315 cm−1), and phosphate (1242–1027 cm−1) groups. These surface functional groups play essential roles in AFB1 adsorption, which is in close agreement with previous works (Peng et al., 2016; Ramales-Valderrama et al., 2016; ZavalaFranco et al., 2018). As shown in Fig. S2 and Table S1 (Supplementary data), in the AFB1-loaded biosorbents, vibrations of these functional groups shifted at lower wavenumbers, and significantly reduced their intensity (relative transmittance), suggesting the participation of these functional groups on AFB1 adsorption.
3.3. Point of zero charge (pHpzc)
Point of zero charge (pHpzc) is a procedure often used for measuring the biosorbent surface charge. All pHpzc values were computed by plotting ΔpH against the initial pH value of the three buffer solutions employed in the sorption experiments. Since similar results were obtained for both doses of biosorbents (0.5% w/v and 0.1% w/v) upon performing different characterization techniques such as pHpzc and zeta potential, most of the results presented herein are those obtained using 0.1% w/v. Lettuce, field horsetail, and Formosa firethorn curves cross the x-axis at average pH values of 5.65, 5.69, and 5.62, respectively. As a result, the surface charge of these biosorbents was zero at those pH values (Fig. 2).
It is well recognized that if pH < pHpzc, the surface of the biomaterial will be positively charged, and if pH > pHpzc, the surface will be negatively charged (Lim et al., 2017). Interestingly, the three tested biosorbents have a high negative-charged surface at the pH value of the intestinal section (pH 7); consequently, at higher doses (0.5% w/v), all of these materials have exceptional AFB1 uptakes (100%) during sorption experiments (Fig. 1, profile a). However, at lower doses (0.1% w/v), lettuce biosorbent had the highest efficiency against AFB1 removal; in these samples, the average biosorption uptake was 95 ± 3% (Fig. 1, profile b).
These results suggest that the interaction type between AFB1 and the biosorbents would be primarily electrostatic in nature, since AFB1 is a very polar molecule with an elevated net positive charge (RamalesValderrama et al., 2016). Furthermore, the extraordinary adsorption property of lettuce biosorbent could be based on a set of chemical and physical mechanisms involving physicochemical interactions and complexation, leading to immobilization of almost all AFB1 molecules.
3.4. Zeta potential (ζ) and electrophoretic mobility
Zeta potential is the electrostatic potential at the electrical double layer surrounding a particle in solution. Undoubtedly, the properties of any interface are significantly influenced by the presence of ionic charges. Fig. 3 (profile a) shows the relationship between zeta potential and pH of the three tested biosorbents. As the pH value increased (up to 7), more negative zeta potential values were recorded. A zeta potential value of − 47 ± 1 mV was observed in Formosa firethorn; however, lettuce and field horsetail biosorbents presented zeta potential values of –32 ± 1.5 mV and − 40 ± 1.5 mV, respectively. Consequently, the high negative-charged surface on these biosorbents – at this particular pH value – results in a high sorption uptake, because of the enhancement of attractive forces between the AFB1 molecules and the biomaterial surface.
To further elucidate this mechanism, a set of experiments with the AFB1 molecule at the different pH values (2, 5, and 7) were also conducted. A strong dependence of electrophoretic mobility on the acidity of the aqueous phase was observed (Fig. 3, profile b). Additionally, a considerable magnitude in the negative surface zeta potential was registered for AFB1 molecules at pH 7 (− 28 ± 4 mV). These results show that the presence of the negative surface charge at the water-non polar AFB1 interface is caused by the hydroxyl ions released by the dissociation-association equilibrium of the water molecules (Marinova et al., 1996). It is well recognized that surrounding the surface of the particle is a layer of ions that have the opposite charge of the particles surface. Thus, in this research, a high positive charge density on the AFB1 surface was observed at neutral pH (Fig. 3, profile b); consequently, higher adsorption uptakes were recorded.
Summarizing, the increase in AFB1 adsorption with an increase in pH can be understood by looking at the charges and changes in the chemical interaction between the AFB1 molecule and the biosorbents. From pHpzc studies, the surface of the three biosorbents was positively charged at pH < 5.6, and negatively charged at pH > 5.6. Thus, clearly at pH < 5.6, both the AFB1 molecule and the biosorbents are positively charged; consequently, minimal absorption would be expected. Interestingly, significant adsorption uptakes occurred at pH 5 (Fig. 1, profile b); this observation suggests that adsorption could be accomplished not only through electrostatic interactions.
3.5. Chlorophylls content
The chlorophyll contents of the biosorbents are shown in Table 2. Lettuce had the highest total chlorophyll content (5.10 mg/g sorbent) when compared with field horsetail (1.32 mg/g sorbent) and Formosa firethorn (3.14 mg/g sorbent). Chlorophyll a (Chl a) and chlorophyll b (Chl b) contents were also significantly different among the biosorbents. Lettuce biosorbent has 1.6-fold and 3.8-fold total chlorophyll than Formosa firethorn and field horsetail, respectively (Table 2).
Various studies have shown that chlorophyll, as well as its watersoluble derivative sodium copper chlorophyllin, can form strong noncovalent complexes in vitro with AFB1 and other polycyclic planar mutagens or carcinogens independent of temperature or pH (Arimoto et al., 1993; Breinholt, Schimerlik, Dashwood, & Bailey, 1995; Hayashi, Schimerlik, & Bailey, 1999; Jubert et al., 2009; Simonich et al., 2007). Consequently, in this research, the formation of a complex with the carcinogen (via electrostatic, π-π orbital interactions and/or hydrogen bonding) may be expected to improve the rate of AFB1 uptake by the biosorbents containing significant amounts of chlorophylls.
In an attempt to confirm these results, another set of laboratory experiments were performed using biosorbents with negligible quantities of chlorophylls. Chlorophylls were extracted from the biosorbents using 96% ethanol, and the chlorophyll-free biomaterials were rinsed three times with deionized water and dried in an oven at 50 °C to constant weight. Adsorption conditions were identical to those described in section 2.3 using 0.1% w/v biosorbent dose at pH 7. The results are shown in Fig. 4. In all cases, AFB1 uptakes were lower than those obtained for pristine biosorbents (Fig. 1, profile b). Lettuce and Formosa firethorn showed moderate AFB1 adsorption capacities, yielding 51% and 53%, respectively. Field horsetail had the lowest biosorption uptake value (37%). These findings support the idea that the adsorption arises by trapping of the AFB1 molecule by chlorophylls through complex formation.
To the best of our knowledge, this is the first in vitro study conducted to evaluate the effectiveness of low contents of biosorbents in removing AFB1 using an in vitro model simulating certain conditions in the gastrointestinal tract of poultry (pH values, temperature, and digestion time). This methodology may not be directly applicable to poultry (due to the fact that it does not use enzymatic activities similar to in vivo environments); however, further in vitro and in vivo studies need to be conducted to prove the effectiveness of these biosorbents in reducing AFB1 without compromising micronutrient bioavailability for poultry, which is undoubtedly the fastest growing and most competitive of all livestock sectors. Research in this direction is in progress in our laboratories.
Mean values ± standard error. Means, within the same column, not sharing a common superscript differ significantly (Tukey p < 0.05).
4. Conclusions
In vitro studies have shown that lettuce – an abundant, inexpensive, and renewable agro-waste material – can be successfully used for the biosorption of AFB1 at low doses (0.1% w/v). Chemical and physical mechanisms including physicochemical interactions such as electrostatic, π-π orbital, hydrogen bonding, and complexation with chlorophylls, are involved in the adsorption process. Therefore, it is expected that lettuce may effectively reduce the bioavailability of AFB1 in in vivo trials.
This article was originally published in Food Chemistry, Volume 345, 30 May 2021, 128863. https://doi.org/10.1016/j.foodchem.2020.128863. This is an Open Access article under the CC BY-NC-ND license (http://creativecommons.org/licenses/by-nc-nd/4.0/).