INTRODUCTION
Commercial poultry production is associated with various stresses decreasing productive and reproductive performance of birds. A growing body of evidence indicates that most of stresses in poultry production at the cellular level are associated with oxidative stress due to excess of free radical production or inadequate antioxidant protection. Recently, a concept of the cellular antioxidant defence has been revised with special attention paid to cell signalling. Antioxidant systems of the living cell are based on three major levels of defence and include several options [1]: Decrease localized oxygen concentration; decrease activity of pro-oxidant enzymes and improve efficiency of electron chain in the mitochondria and decreasing electron leakage leading to superoxide production; prevention of chain initiation by scavenging initial radicals due to inducing various transcription factors (e.g., Nrf2, NF-κB and others) and ARE-related synthesis of AO enzymes (SOD, GSH-Px, CAT, GR, GST, etc.); binding metal ions (metal-binding proteins) and metal chelating; decomposition of peroxides by converting them to non-radical, nontoxic products (SeGSH-Px); chain breaking by scavenging intermediate radicals such as peroxyl and alkoxyl radicals (vitamins E, C, GSH, uric acid, ubiquinol, bilirubin, etc.); repair and removal of damaged molecules (methionine sulfoxide reductase, DNA-repair enzymes, chaperons, etc.) and vitagene activation and synthesis and increased expression of protective molecules (GSH, Thioredoxins, SOD, HSPs, sirtuins, etc.)
Indeed, understanding roles of vitagenes in stress resistance of poultry as a background for the development of effective strategies to deal with stresses is an emerging topic of research [1-5]. It is known that vitagenes are responsible for synthesis of various protective molecules and HSP synthesis is under vitagene control. However, by the time of writing no comprehensive review on the roles and effects of HSPs in poultry biology has appeared. Therefore, the aim of this review is a critical analysis of the role of HSPs in poultry biology with special emphasis to their functions as an essential part of the vitagene network, responsible for adaptive ability of the cells or whole organisms to various stress conditions.
1. Heat Shock Response and Heat Shock Factors
The heat shock response (HSR) is one of the main adaptive stress responses of the cell, restoring cellular homeostasis upon exposure to proteotoxic stress, including heat shock, cold, oxidative stress, hypoxia, toxins, chemicals, pathogen, etc. (Table 1) [6-8]. In fact, cooperative interactions between the transcription factors and various homeostatic mechanisms are responsible for effective adaptation to stressful conditions [9-11]. Indeed, to maintain vital life function it is imperative that organisms preserve the integrity of their proteins. Therefore, HSR in vertebrates is characterized by the induction of HSPs and related elements, such as the ubiquitin–proteasome system [7]. Because HSPs act as molecular chaperones that facilitate protein folding and suppress protein aggregation, this response plays a major role in maintaining protein homeostasis. Generally, HSR is regulated mainly at the level of transcription by four heat shock transcription factors (HSFs), including HSF1, HSF2, HSF3, and HSF4, which bind to HSE [9], thus resulting in stimulation of HSPs expression.
Among other heat shock factors, HSF1 has received tremendous attention as the main factor governing the HSR by coordinating stress-induced transcription [12]. Although originally discovered as a response to thermal stress, HSR can be triggered by a variety of stress conditions that interfere with protein folding and result in accumulation of misfolded or aggregated proteins [13]. HSF1 activation is a multistep process that is negatively regulated by chaperones, including HSP90 and HSP70 [14], which sets the stage for rapid induction of gene expression within minutes of cellular stress [15]. In physiological conditions the majority of HSFs form a complex with HSP70 or HSP90 interacting with the HSF1 activation domain. In stress conditions, HSP70 and HSP90 form complexes with denatured proteins, which releases HSFs [16]. Furthermore, in unstressed state, HSF1 is present in the cytoplasm as a latent monomeric molecule. Upon heat shock, monomeric HSF1 is hyperphosphorylated and converts to a trimer with the capacity to bind DNA that accumulates in the nucleus and subsequently binds to the heat shock element within the promoter region of HSP genes. In addition, extensive posttranslational modifications such as phosphorylation, acetylation, and sumoylation are thought to fine-tune HSF1 activity [8, 11]. The increased expression of HSPs continues until the amount of HSP70 and HSP90 reaches the level sufficient to block the activation domain of the HSFs [16].
2. Chicken HSF
Avian cells express at least three HSFs (HSFs 1-3). Initially, three avian HSF genes corresponding to a novel factor, HSF3, and the avian homologs of mammalian HSF1 and HSF2 have been cloned [17[. The predicted amino acid sequence of HSF3 is approximately 40% related to the sequence of HSF1 and HSF2. Similar to HSF1 and HSF2, the HSF3 message, is coexpressed during developmen
Table 1. Chemical and physical inducers with their target genes in the stress response pathway (Adapted from [3, 63, 334])
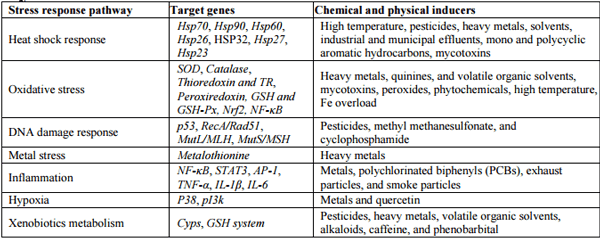
In most tissues, which suggests a general role for the regulatory pathway involving HSF3 [17]. It was shown that the regulatory domain is located between the transcriptional activation domains and the DNA binding domain of HSF1 and is conserved between mammalian and chicken HSF1 but is not found in HSF2 or HSF3 [18]. Indeed, the regulatory domain was found to be functionally homologous between chicken and human HSF1. In fact, HSF3 is negatively regulated in avian cells and acquires DNA-binding activity in certain cells upon heat shock [19]. Induction of HSF3 DNA-binding activity is delayed compared with that of HSF1 and heat shock leads to the translocation of HSF3 to the nucleus [19]. It has been shown that HSF1 is rapidly activated by even mild heat shock, while HSF3 is activated only by severe heat shock. In contrast, HSF2 is not activated by heat stress and has been speculated to have developmental functions [20]. Indeed, cHSF3 (chick HSF3) was activated at higher temperatures than the cHSF1. In fact, at a mild heat shock, such as 41oC, only cHSF1 was activated, whereas both cHSF1 and cHSF3 were activated following a severe heat shock at 45oC. Similarly, cHSF3 was activated by treating cells with higher concentrations of sodium arsenite compared to cHSF1. Furthermore, the DNA binding activity of cHSF3 by severe heat shock lasted for a longer period than that of cHSF1. In addition, the total amount of cHSF3 increased only upon severe heat shock, whereas that of HSF1 decreased. Indeed, cHSF3 is involved in the persistent and burst activation of stress genes upon severe stress in chicken cells [20]. It seems likely that denaturation of nascent polypeptides could be the first trigger for the activation of cHSF1 and cHSF3 [20]. It has been suggested that HSF3 has a dominant role in the regulation of the heat shock response and directly influences HSF1 activity. Thus, disruption of the HSF3 gene results in the severe reduction of heat shock gene expression and loss of thermotolerance [21]. In addition, null cells lacking HSF3, yet expressing normal levels of HSF1, exhibited a severe reduction in the heat shock response, as measured by inducible expression of heat shock genes, and did not exhibit thermotolerance.
Important information related to HSFs in avian species has been obtained in experiments with chick embryos. In fact, it was shown that HSF3 was almost constantly expressed in various tissues during early to late chicken embryonic development [22]. The expression of HSF1 was equally high in most tissues early in development and thereafter declined to different levels in a tissue-dependent manner and HSF3 became the dominant heat-responsive factor mediating stress signals to heat shock gene expression in the chicken. Furthermore, the high-level and ubiquitous expression of HSF2 as well as HSF1 and HSF3 in early embryogenesis suggest the involvement of these factors in all developmental processes [22]. It is interesting to note that in avian, HSF1 and HSF3 are maintained in a cryptic monomer and dimer form, respectively, in the cytoplasm in the absence of stress. Upon heat stress, they undergo conformational change associated with the formation of a trimer and nuclear translocation and the nuclear localization signal acts positively on the trimer formation of cHSF3 upon stress conditions [23]. Indeed, avian cells express two redundant heat-shock responsive factors, HSF1 and HSF3, which differ in their activation kinetics and threshold induction temperature. For example, in birds, HSF1 only slightly induces HSP70 expression during heat shock and indeed HSF3 is a master regulator of the heat shock genes in avian cells, as is HSF1 in mammalian cells [24]. Avian cells lacking two heatinducible HSFs, HSF1 and HSF3 were generated [25]. In addition to complete loss of activation of heat shock genes under stress conditions, these cells exhibited a marked reduction in HSP90α expression under normal growth conditions. Reduction in HSP90α expression caused instability of a cyclin-dependent kinase, Cdc2, and cell cycle progression was blocked mainly at the G2 phase, but also at G1 phase even at mild heat shock temperatures. Restoration of HSP90α expression rescued the temperature sensitivity without induction of HSPs [25].
Whereas HSF1 mediates transcriptional activity only in the brain upon severe heat shock, HSF3 is exclusively activated in blood cells upon light, moderate, and severe heat shock, promoting induction of heat-shock genes [26]. Although not activated, HSF1 is expressed in blood cell nuclei in a granular appearance, suggesting regulation of genes other than heat-shock genes. It was shown that HSF1 and HSF3 mediate transcriptional activity of adult tissues and differentiated cells in a nonredundant manner. Instead, an exclusive, tissuespecific activation is observed, implying that redundancy may be developmentally related [26].
The heat shock response regulated by the HSF family is shown to consist of the induction of classical as well as of nonclassical heat shock genes, both of which might be required to maintain protein homeostasis [9]. Recently, additional information on the roles of HSF2 has been obtained. In particular it has been shown that vertebrate HSF2 is activated during heat shock in the physiological range [27].
HSF2 deficiency reduces threshold for chicken HSF3 or mouse HSF1 activation, resulting in increased HSP expression during mild heat shock. HSF2-null cells are more sensitive to sustained mild heat shock than wildtype cells, associated with the accumulation of ubiquitylated misfolded proteins. Furthermore, loss of HSF2 function increases the accumulation of aggregated polyglutamine protein and shortens the lifespan of R6/2 Huntington's disease mice, partly through αB-crystallin expression [27]. In fact, HSF2 was identified as a major regulator of proteostasis capacity against febrile-range thermal stress [27]. It was also shown that chicken HSF3, but not chicken HSF1, induces the expression of the major avian pyrogenic cytokine IL-6 during heat shock [28].
In general, important roles of HSFs in adaptation of poultry to various stress conditions are difficult to overestimate. However, recent genome-wide studies have revealed that HSF1 is capable of reprogramming transcription more extensively than previously assumed; it is also involved in a multitude of processes in stressed and non-stressed cells [29].
3. Heat Shock Proteins
Heat shock proteins (HSPs) are highly conserved families of proteins discovered in 1962 [30]. Later, it has been realized that most HSPs have strong cytoprotective effects and are molecular chaperones for other cellular proteins. Taking into account current knowledge of the mode of action of HSPs, the name of ‘‘stress proteins’’ would be more appropriate for them but due to historical reasons they are still called HSPs. Indeed, in the case of oxidative stress, HSP network participates in detecting intracellular changes, protecting against protein misfolding and preventing activation of downstream events related to inflammation and apoptosis (Figure 1)[31]. Since oxidative stress plays a major role in a number of diseases and disease mechanisms in human [31] and decreases productive and reproductive performance in farm animals [32], it is likely that any medication/treatment that is able to reduce levels of oxidative stress will make a significant impact on human health and animal performance. Some HSPs are constitutively expressed, whereas others are strictly stress-inducible. Under physiologic conditions, HSPs play an important role as molecular chaperones by promoting the correct protein folding and participating in the transportation of proteins across intracellular membranes and repair of denatured proteins. Therefore, HSPs participate in the regulation of essential cell functions, such as protein translocation, refolding, assembly and the recognition, prevention of protein aggregation, renaturation of misfolded proteins, degradation of unstable proteins, etc. [33]. It should be mentioned that the events of cell stress and cell death are linked and HSPs induced in response to stress appear to function at key regulatory points in the control of apoptosis [34-35]. A key feature of HSPs is their ability to provide cytoprotection. Synthesis of these proteins under stress conditions is a highly conserved mechanism of the cell response and adaptation is common among all living organisms (Figure 2). In fact, HSPs are synthesized in response to a great variety of cellular stresses, including heat stress, hypoxia, ischemia, hypothermia, virus infections as well as the effects of various toxicants, including mycotoxins [7]. It is important to note that upregulation of the synthesis of HSPs is considered an endogenous adaptive phenomenon leading to improved tolerance to various stress conditions/factors. In mammals and birds the HSP superfamily includes five broadly conserved families of proteins (Table 2). Among them HSP70, HSP90 and HSP32 (HO-1) are considered as vitagenes.
3.1 HSP70
Among the HSPs, HSP70 is one of the most conserved and important protein families and has been extensively reviewed [36-39] and will be briefly dealt with.
Figure 1. Functions of HSP under physiological and stress conditions (Adapted from [337])
Figure 2. Activation of the HSP by specific stimuli and their protective effects (Adapted from [33])
Table 2. Mammalian heat shock proteins (Adapted from [335-336])
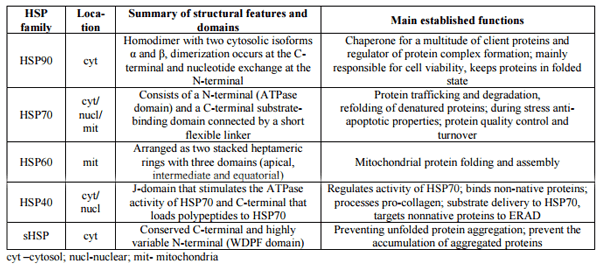
Here. In fact, HSP70 refers to a family of 70 kDa chaperone proteins. Some of the important house-keeping functions attributed to HSP70 include [40]: import of proteins into cellular compartments; folding of proteins in the cytosol, endoplasmic reticulum and mitochondria; degradation of unstable proteins; dissolution of protein complexes; control of regulatory proteins; refolding of misfolded proteins and translocation of precursor proteins into mitochondria. These molecular chaperones are implicated in a wide variety of cellular processes, including protein biogenesis, protection of the proteome from negative consequences of stress, recovery of proteins from aggregates, facilitation of protein translocation across membranes, as well as disassembly of particular protein complexes and cell signaling for growth, differentiation, and apoptosis [41]. In particular, HSP70 can inhibit apoptosis by interfering with target proteins [42]. In eukaryotic cells, HSP70s are subject to a large number of post-translational modifications [36]. These ATP-dependent chaperones represent central components of the cellular protein surveillance network and are involved in a large variety of protein-folding processes. In fact, they effectively interact with practically all proteins in their unfolded, misfolded, or aggregated states but do not interact with their folded counterparts [36]. A number of eukaryotic proteins are regulated through transient association with HSP70, including steroid hormone receptors, kinases and transcription factors. Eight different and unique HSP70 have been identified in eukaryote cells being distributed in different subcellular compartments, including cytosol, nucleus, endoplasmic reticulum, and mitochondria [43- 44]. The two most important members of the HSP70 family are the constitutively expressed 73 kDa heat shock cognate (HSC73, HSC70, HSPA8) and stress-inducible 72 kDa heat shock protein (HSP72, HSP1A) [45]. Indeed, under normal conditions HSP70 proteins function as ATP-dependent molecular chaperones maintaining important cell functions related to proteostasis [36]. Under various stress conditions additional synthesis of stress-inducible HSP70 enhances the ability of stressed cells to deal with increased concentrations of unfolded or denatured proteins [41]. HSP70 expression is associated with a reduction in JNK1 phosphorylation and/or an increase in oxidative capacity consequential of improvements in mitochondrial homeostasis [46]. It seems likely that HSP70s do not work alone but with a team of cochaperones. Recently it has been found that the organelle distribution of HSP70 is determined by their specific lipid compositions. In particular, HSP70 attach to lipids by extended phospholipid anchorage, with specific acidic phospholipids associating with HSP70 in the extended conformation with acyl chains inserting into hydrophobic crevices within HSP70, and other chains remaining in the bilayer [44]. It seems likely that this could represent an important connection between HSPs and lipid quality control in the cell and the HSP90/HSP70-based chaperone machinery may function as a comprehensive protein management system for quality control of damaged proteins. Actually, in a recently developed model, it was proposed that the heat shock protein HSP90/HSP70-based chaperone machinery played a major role in determining the selection of proteins that have undergone oxidative or other toxic damage for ubiquitination and proteasomal degradation [47].
3.2 Chicken HSP70
In 1978 it was shown that the pattern of proteins synthesized by chicken embryo fibroblasts changes dramatically after heat treatment (45oC for a few hours). In fact, three proteins (Mr = 22,000, 76,000, and 95,000) accounted for almost 50% of the cell's protein synthetic capacity immediately after the heat-shock [48]. The university of the heat shock response and conservation of proteins induced by this type of stress was proven in different experimental conditions. In particular, antibodies to chicken HSPs, cHSP89 and cHSP70, cross-reacted with proteins of similar molecular weights in embryonic and adult chicken tissues and in extracts from widely different organisms ranging from yeast to mammals [49]. Heat-shock polypeptides of identical sizes of 85,000, 70,000, and 25,000 Da were synthesized predominantly in chicken embryo fibroblasts and in many different organs of 18-day-old embryos at 42.5-44°C [50]. Effects of heat treatments on chick embryo fibroblasts, Drosophila embryonic cells, and human lymphoblastoid cells have been compared [51]. Cells from all three species synthesize large HSPs with Mr = 70,000 and 84,000- 85,000. Different small HSPs with Mr between 22,000 and 27,000 are made at high rates in heat-treated chicken and Drosophila cells but could not be observed in human cells. It was found that chicken reticulocytes respond to elevated temperatures by the induction of only one heat shock protein, HSP70, whereas lymphocytes induce the synthesis of all four heat shock proteins (HSP89, HSP70, HSP23 and HSP22). The synthesis of HSP70 in lymphocytes was rapidly induced by small increases in temperature (2-3 °C) and blocked by preincubation with actinomycin D [52]. Furthermore, incubation of chicken reticulocytes at elevated temperatures (43-45°C) resulted in a rapid change in the pattern of protein synthesis, characterized by the decreased synthesis of normal proteins, e.g., alpha and beta globin, and the preferential and increased synthesis of HSP70 [53]. Indeed, the rapid 20-fold increase in the synthesis of HSP70 was observed after heat shock and preincubation of reticulocytes with the transcription inhibitor actinomycin D or 5,6-dichloro- 1-beta-D-ribofuranosylbenzimidazole blocked the heat shock-induced synthesis of HSP70.
In 1986 Morimoto and co-authors studied organization, nucleotide sequence, and transcription of the chicken HSP70 gene. They isolated a gene encoding a 70,000-Da heat shock protein (HSP70) from a chicken genomic library and showed that the order and spacing of the sequences share many features in common with the promoter for the human HSP70 gene [54]. The expression of HSP70 during maturation of avian erythroid cells was also studied [55]. It was shown that definitive red cells respond to heat shock by a 10- to 20-fold increase in HSP70 protein synthesis with little change in HSP70 mRNA levels. Therefore, the increased expression of HSP70 in cells was due to increased translatability of HSP70 mRNA. Furthermore, the authors showed that HSP70 expression in erythroid cells is lineage specific and although HSP70 was constitutively expressed, neither HSP70 synthesis nor HSP70 mRNA levels were heat shock inducible in primitive red cells.
HSP70 was shown to constitutively expressed in the embryonic chicken lens. In fact, HSP70 mRNA in the embryonic chicken lens was associated primarily with cells in the early stages of fiber formation, and increased transcription of this gene was part of the differentiation process [56]. It was shown that the heat induced increase in HSP70 mRNA and protein in broiler liver, in vivo, are time dependent, similar to that in mammals [57]. An increase in the amount of HSP70 was detected from the first up to the fifth hour of acute heat exposure (35°C for 5 h), while an increase in HSP70 mRNA peaked at 3 h. It seems likely that heat shock response in avian species is related to temperatures above 41°C. For example, the spatial expression of HSP70 transcripts was detected in chicken embryos under normal incubation conditions and moderate heat stress (41°C) did not induce enhancements on HSP70 mRNA levels [58]. At the same time, acute exposure to severe heat stress (44°C) for one hour resulted in a fifteen-fold increase in HSP70 mRNA levels. It is interesting to note that the return of stressed embryos to normal incubation temperature resulted in increased HSP70 mRNA levels for three hours which was normalised after six hours. The increased expression of HSP70 in broiler chicken embryos was shown to be affected not only by heat (40°C) but also by cold (32°C) stress, and is tissue- and age-dependent [59]. In fact, HSP70 was detected in the liver, heart, breast muscle, and lungs and the brain contained 2- to 5-times more HSP70 protein compared to the other embryonic tissues. These data are in agreement with our observations indicating low level of vitamin E and high levels of PUFAs in chicken embryonic brain [60]. Therefore, increased HSP70 expression is an adaptive mechanism of increasing antioxidant defences. Younger embryos had higher HSP70 synthesis than older embryos, irrespective of the type of thermal stressor [59]. Again, these data confirm our finding about maturation of the antioxidant defences during chicken embryonic development [61].
It was shown that HSP70 expression in postnatal chickens is tissue- and allele-dependent [62]. Indeed, the expression of HSP70 gene in the liver was significantly (more than 2-fold) higher than that in the muscle under normal growth conditions. This could reflect an importance of HSP70 chaperone functions, since the liver is the major site of synthesis of many important proteins. However, during acute heat stress (44oC for 4 hours) the expression of HSP70 gene in the brain was the highest being significantly different from those in the liver and muscle. This adaptive response by HSP70 also is an important mechanism to compensate for relatively low levels of antioxidants in the brain tissue of the chicken [63]. Long-term, moderate heat stress (30-32oC) was associated with significantly increased HSP70 levels in mononuclear blood cells of laying hens [64]. However, the age-dependent responses of different genotypes were not uniform. HSP70 gene expression was genderdependent with significantly higher levels in male than in female chickens [65] and tissue-dependent heat induction of HSP70 expression may correlate with DNA methylation pattern in the HSP70 promoter [66]. During the exposure to heat stress (37±1 oC), the heart, liver and kidney of broiler chickens exhibited increased amounts of HSP70 protein and mRNA. The expression of HSP70 mRNA in the heart, liver and kidney of heat-stressed broilers increased significantly and attained the highest level after a 2-h exposure to elevated temperatures. Significant elevations in HSP70 protein occurred after 2, 5, and 3 h of heat stressing, respectively, indicating that the stress-induced responses vary among different tissues [67]. Furthermore, the expression of HSF3 and HSP70 mRNA in Lingshan chickens (LSC) and White Recessive Rock (WRR) exhibited species-specific and tissuespecific differences during heat treatment [68]. For example, after 2 h of heat treatment, HSP70 expression was significantly higher in the liver and leg muscle of WRR compared to LSC. Recent analysis of genetic diversity of the HSP70 gene in 8 native Chinese chicken breeds indicates presence of 36 variations, which included 34 single nucleotide polymorphisms and 2 indel mutations [69]. Furthermore, 57 haplotypes were observed, of which, 43 were breed-specific and 14 were shared.
HSP expression in the gut could be considered as an important mechanism of the antioxidant protection [5]. However, there were no effects of HSP70 overexpression on intestinal morphology under heat stress, but there was a strong correlation between HSP70 expression and the digestive enzyme activity in broilers [70]. In another study from the same department, HSP70 induction was shown to protect the intestinal mucosa from heat-stress injury by improving antioxidant capacity of broilers and inhibiting the lipid peroxidation production [71]. In fact, HSP70 significantly protected the integrity of the intestinal mucosa from heat stress (36 ± 1°C) by significantly elevating antioxidant enzyme activities (SOD, GSH-Px and total antioxidant capacity) and inhibiting lipid peroxidation to relieve intestinal mucosal oxidative injury.
To investigate the alterations introduced by domestication and selective breeding in heat stress response, two experiments were conducted using Red Jungle Fowl (RJF), village fowl (VF), and commercial broilers (CB). Birds of similar age (30 d old) or common body weight (930 ± 15 g) were exposed to 36 ± 1°C for 3 h [72]. The RJF at a common age and common BW showed significantly higher levels of basal HSP70 and cortisone compared with VF and CB. Heat treatment was shown to significantly increase body temperature, heterophil: lymphocyte ratio, and plasma corticosterone concentration in CB but not in VF and RJF. Irrespective of stage of heat treatment, RJF showed lower heterophil:lymphocyte ratio and higher plasma corticosterone concentration than VF and CB. It was concluded that domestication and selective breeding are leading to individuals that are more susceptible to stress rather than resistant [72]. Furthermore, laying hens exposed to HS (32.6°C) showed higher concentrations of HSP70 in the liver [73]. In addition, kind gentle hens (a line of group-selected hens for high productivity and survivability) had higher concentrations of HSP70 than DeKalb XL hens (commercial line of individually selected hens for high egg production) regardless of treatment.
HSP70 is also shown to be expressed in other avian species. Notably, quail HSP70 showed 98% homology with HSP70 stress protein in Gallus gallus and 99% homology with Numida meleageris [74]. Duck HSP70 gene was also identified and characterised (GenBank: EU678246) and shown to contain no introns [75]. Fifteen variations were identified within the open reading frame. The expression of duck HSP70 gene was tissue-specific and the highest expression level was seen in pectoral muscle [75]. To sum up, the results from the aforementioned studies consistently demonstrate that increased HSP70 expression in chicken tissues is one of the most important protective responses to prevent or deal with, detrimental changes in protein structure and functions due to various stresses. However, there is a need for further research to understand molecular mechanisms of HSP70 regulation in avian species.
3.3 HSP90
Hsp90, the major soluble protein of the cell, has recently received great attention and a range of reviews described its structure, functions and regulation [76-79]. In fact, in the cell, HSP90 is known to comprise 1-2% of total proteins under non-stress conditions and it is further upregulated under stress [80]. For example, heat shock (37–42°C) has been reported to induce HSP90 levels by as much as twofold [81]. Furthermore, fish naturally living in a hot spring with relatively high water temperature (34.4±0.6°C) is characterised by increased levels of all the studied HSPs (HSP70, HSP60, HSP90, HSC70 and GRP75) compared with fish living in normal river water temperature [82].
HSP90 is expressed as a 90 kDa protein and its functional molecule is a homodimer (α/α or β/β) and each monomer consists of three domains. They are NH2- terminal nucleotide binding domain (a binding site for ATP/ADP), the middle domain (the binding site for nuclear localization signal and client proteins) and the Cterminal domain (the site of dimerization and cochaperone binding) [83-84]. The HSP90 family in mammalian cells consists of four major homologs including two cytoplasmic isoforms HSP90α (inducible form) and HSP90β (constitutive form) [85], HSP90B located in endoplasmic reticulum and tumor necrosis factor receptor-associated protein (TRAP) found in mitochondria and the inner membrane space [86] (Table 3). It is interesting to note that HSP90α and HSP90β share 86% amino acid identity and are expressed in all nucleated cells.
Table 3. Isoforms of HSP90 (Adapted from [86])
HSP90 is a highly efficient, ATP-dependent molecular chaperone involved in the maturation and stabilisation of a wide-range of proteins in both physiological and stress conditions being an important hub in the protein network that maintains cellular homeostasis and function [87]. HSP90 belongs to a family of proteins known as "chaperones," which are solely dedicated to helping other proteins (client proteins) correct folding, function and stability. Indeed, cellular stress causes protein denaturation, and they cannot function properly and must be repaired or eliminated with the help of chaperones [88].
HSP90 deals with more than 200 important clients which are involved in signal transduction, including many steroid hormone receptors, receptor tyrosine kinases, Src family members, serine-threonine kinases, cell cycle regulators, telomerase and many other proteins (Figure 3) [84, 89-90]. It is difficult to overestimate chaperoning functions of HSP90 related to various nuclear proteins regulating DNA replication, DNA repair, DNA metabolism, RNA transcription and RNA processing [84] and the protective action of HSP90 is related to posttranslational modifications of soluble nuclear factors as well as histones [76]
Figure 3. HSP90 interactions with client proteins (Adapted from [76])
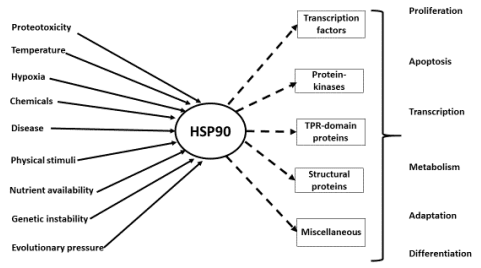
It was suggested that HSP90 clients are associated with major physiological events including signal transduction, cell cycle progression, transcriptional regulation, natural and acquired immunity and intracellular movement of proteins [84, 91]. In fact, HSP90 participates in many cellular processes including cell cycle control, cell survival, hormone and other signaling transduction pathways, often acting as hormone receptors and is considered to be key player in maintaining cellular homeostasis and adaptive response to stress [87]. In many cases, HSP90-assocoated stress response is orchestrated via HSF1, which under stress conditions upregulates several hundred genes including HSP90. It is known that under physiological condition, as a client protein, HSF1 is kept in an inactive monomeric form through the transient interaction with Hsp90 [84]. During stress, HSF1 dissociates from HSP90, homotrimerizes, undergoes phosphorylation and translocates to the nucleus to perform its gene-expression regulatory functions [84]. As a matter of fact, HSP90 is regulated transcriptionally through direct interactions with the transcription factor HSF [92]. Generally, HSP90 is present in cells in equilibrium between a low chaperoning activity ‘latent state’ in physiological conditions and an ‘activated state’, with increased chaperoning efficiency in stress conditions [93]. HSP90 usually works as a complex with other chaperones and over 20 co-chaperones [94] and increased expression of HSP90 have been shown to be associated with the tolerance of hypothermia, cell proliferation, and cell cycle control [95]. In fact, cochaperones assist HSP90 in its conformational cycling, act as substrate recognition proteins and provide additional enzymatic activity [96] . It seems likely that under heat stress conditions, co-chaperones allow HSP90 to prevent aggregation of unfolded proteins [12]. Indeed, HSP90 involves in the folding, stabilization, activation and assembly of its client proteins through the formation of complexes with co-chaperones such as HSP70, HSP40, Hop, Hip and p23 [97].
The molecular chaperones HSP90 and HSP70 form a multichaperone complex, in which both are connected by a third protein called Hop. Indeed, Hop (HSP70/HSP90 organizing protein) facilitates interaction between HSP90 and HSP70 helping substrate to be efficiently transferred from HSP70 to HSP90 [98]. It seems likely that the interplay between the two chaperone machineries affecting the trafficking and turnover of several hundred signaling proteins as well as removal of damaged and aberrant proteins via the ubiquitinproteasome pathway is of great importance for cell viability and adaptability. HSP90 is shown to possess an ATPase activity, which is known to be essential to modulate the conformational dynamics of the protein. In fact, ATP hydrolysis is associated with the HSP90 dimer transitioning into its ‘‘open’’ conformation and releasing the client protein [91]. The system is regulated by posttranslational modifications including phosphorylation, acetylation, nitrosylation and methylation and uses a range of co-chaperones mediating interactions with HSP90 client proteins [84, 87]. Therefore, HSP90 has been considered to be a key factor at the crossroads of genetics and epigenetics [76]
3.4 Chicken HSP90
A cDNA clone for the 90kDa heat-shock protein was isolated by direct immunological screening of a chicken smooth muscle cDNA expression library [99]. It was shown that HSP90 is increased in heat-shocked chick embryo fibroblasts [100]. Furthermore, HSP90 from chicken liver has been purified and physically characterised [101]. The protein was shown to be an elongated dimer with a molecular weight of 160,000 and a frictional ratio of 1.6, extensively phosphorylated and partitioned totally into the aqueous phase. A comparison of the amino acid sequence of the chick HSP90 to that of the homologous HSP90 from yeast to man, reveals 64- 96% identity respectively [102]. The authors suggested that two hydrophilic regions A and B may play a role in the interaction of HSP90 with other proteins such as steroid hormone receptors. In fact, the dimeric form of the HSP90 was confirmed and its structure was shown to be stabilized by hydrogen bonds [103]. Furthermore, the cDNA-derived amino acid sequence of chick HSP90 revealed a "DNA like" structure: potential site of interaction with steroid receptors [102]. The nucleotide sequence of a 2652 bp derived from a chicken HSP90 genomic clone was reported and two introns have been identified [104]. It was proven that HSP90 gene expression is constitutive and heat inducible. In the chick oviduct cells, HSP90 was located in the cytoplasm as aggregates, often inside small vesicles, while in the apical part of the cell, HSP90 was located at the Golgi complex [105]. The epithelium also exhibited some cells with high levels of HSP90. It is interesting to note that HSP90 is associated with both microtubules and microfilaments [106]. In fact, C-terminal half of HSP90 contains a sequence which is responsible for the cytoplasmic localization of the protein and the cytoplasmic anchoring signal is located between amino acids 333 and 664 [107].
It was shown that in contrast to HSP70, the 35S metabolically-labelled HSP90, which accumulates in the cytosoluble fraction 6 - 8 h after serum treatment, is not preferentially translocated to the nuclear compartment, although a small fraction is always present in the nucleus [108]. It was also demonstrated that serum- or insulininduced accumulation of HSP90α mRNA results from an activation of gene transcription and that hsp90α promotor activity is induced approximately fivefold after serum stimulation. Therefore, chicken HSP90 constitutively expressed in most cells, is up-regulated by thermal stress and by developmental and mitogenic stimuli. Indeed, a transient induced expression of the HSP90α gene takes place at both the messenger RNA and the protein synthesis level. This response is protein synthesis dependent and DNA synthesis independent. A possible link between cell cycle and HSP90α regulation was suggested [109]. It seems likely that the HSP90 alpha and beta genes are the result of a gene duplication event that occurred at the time of the emergence of vertebrates [110]. Furthermore, avian HSP90β mRNA is not inducible by thermal stress or mitogenic stimuli, contrary to the mouse and human HSP90 alpha and beta mRNAs. Indeed, chicken HSP90β is the only vertebrate HSP90 insensitive to heat shock and there are some specific features of HSP90 beta gene structure and location explaining why chicken HSP90 beta mRNA is generally less abundant than alpha and is not inducible by heat shock or serum/growth factor stimulation [111].
The importance of ATP binding and hydrolysis by HSP90 in formation and function of protein heterocomplexes was shown [112]. Chicken HSP90 hydrolysing ATP activity was found to be 10-100-fold lower than that in yeast HSP90 and TRAP1, an HSP90 homologue found in mitochondria [113]. The authors showed that sequences within the last one-fourth of HSP90 regulate ATP hydrolysis. The N-terminal ATP binding domain of HSP90 is necessary and sufficient for interaction with estrogen receptor [114].There are two sites in HSP90 binding ATP. In fact, HSP90 N-terminal domain has a nonconventional nucleotide binding site and HSP90 possesses a second ATP-binding site located on the C-terminal part of the protein [115]. HSP90 chaperone activity was shown to require the full-length protein and interaction among its multiple domains, indicating that the cooperation of multiple functional domains is essential for active, chaperone-mediated folding [116].
The expression of HSP90 increased in the heart, liver and kidney of broilers after exposure to increased temperature for 2 h [117]. In the heart and kidney, HSP90 mRNA transcription levels exhibited the same trend as the protein expression of HSP90. Induction of HSP90 mRNA and HSP90 protein at an early stressing stage indicated that heat stress can directly stimulate and quickly initiate the transcription of HSP90 mRNA and translation of HSP90 protein to protect cells. The HSP90α gene is shown to play an evolutionarily conserved role during somitogenesis in vertebrates in addition to providing protection to all cells of the embryo following stress [118].
It was suggested that HSP90 can participate directly in the function of a broad range of cellular signal transduction components, including retinoid receptor signal transduction [119]. In eukaryotic cells, HSP90 is associated with several protein kinases and regulates their activities. HSP90 was also reported to possess an autophosphorylase activity [120]. In fact, chicken HSP90 participates in folding and stabilization of signaltransducing molecules including steroid hormone receptors and protein kinases and both amino- and carboxyl-terminal domains of HSP90 interact to modulate chaperone activity [121]. Depletion of HSP90β induces multiple defects in B cell receptor signaling [122]. Indeed, inhibition of HSP90 with geldanamycin resulted in the inactivation of MAPK/ERK and PI3K/AKT pathways leading to significantly reduced levels of IFN-γ, IL-6 and NO mRNAs in avian macrophages [123].
Therefore, in contrast to mammals, HSP90α but not HSP90β may play a major role in CpG ODN(2007) induced immunoactivation in avian macrophage cells. Collectively, these observations strongly suggest that signaling roles of HSP90 in avian species need further investigation. Recently, four novel members of the 90 kDa heat shock protein (HSP90) family expressed in Japanese quail, Coturnix japonica have been described [124].
The coding regions of the genes, CjHSP90AA1, CjHSP90AB1, CjHSP90B1 and CjTRAP1, exhibited more than 94% similarity to their related genes in chicken. Furthermore, CjHSP90AA1 exhibited a robust response to heat shock treatment.
3.5 HSP32 (HO-1)
HO-1 is the stress-inducible isoform of the three HO isoforms described to date, serving as a critical protective mechanism in vertebrate systems responsible for adaptation to oxidative, inflammatory, and cytotoxic stress [125-126]. In fact, HO-1 (32 kDa), also known as heat shock protein-32 (HSP32), is shown to be expressed at a relatively low level in most tissues. It is proven that HO-1 is endoplasmic reticulum phase II enzyme catalysing the rate-limiting step in heme degradation, producing free iron (Fe2+), carbon monoxide (CO) and biliverdin [127]. Biliverdin is subsequently reduced to bilirubin by biliverdin reductase. It is interesting to mention that the products of the aforementioned reaction can trigger signalling cascades leading to improvement of antioxidant defences and protection against oxidative stress. In particular, CO can modulate the production of proinflammatory or anti-inflammatory cytokines and mediators having immunomodulatory effects with respect to regulating the functions of antigen-presenting cells, dendritic cells, and regulatory T cells [128]. It seems likely that products of the HO-1 reaction namely CO and biliverdin have also cytoprotective, anti-inflammatory and anti-apoptotic properties in stress conditions [129-131]. Cells exposed to low concentrations of CO were shown to respond by an increase in ROS formation (e.g. oxidative conditioning) with important consequences for inflammation, proliferation, mitochondria biogenesis, and apoptosis [132]. Actually, the degradation of heme by HO-1, the signaling actions of CO, the antioxidant protective action of biliverdin/bilirubin, and the sequestration of Fe2+ by ferritin are suggested to contribute to the anti-inflammatory effects of HO-1 [133] and increase stress resistance. Furthermore, recent studies have demonstrated that HO-1 inhibits stress-induced extrinsic and intrinsic apoptotic pathways in vitro [134]. The vital importance of HO-1 in stress adaptation have been confirmed in HO-1-deficient mice models showing atypical proinflammatory immune response [135] with increased vulnerability to endotoxin sepsis [136], defective expression of interferon-β [137] and increased susceptibility to apoptosis [138-139]. Morover, HO-1 knockout mice were characterised by very low survival (~1%–5% of litters) and high levels of oxidative stress with a shortened life span [140]. In fact, HO-1 knockout mice were shown to be extremely sensitive to oxidative stress caused by ischemia and reperfusion [141-142] and to develop anemia associated with hepatic and renal iron overload leading to oxidative tissue injury and chronic inflammation [136]. The aforementioned observations provide substantial evidence to support the implication of HO-1 in stress response.
The half-lives of HO-1 mRNA and protein are shown to be approximately 3 hours and 15–21 hours, respectively [143]. In humans, the HO-1 gene (Hmox1) is located on chromosome 22q12 and consists of four introns and five exons. The regulatory region of the mammalian HO-1 gene has a promoter, a proximal enhancer, and two or more distal enhancers (for review see [144]). The Hmox1 promoter is shown to exhibit a range of binding sites (for AP-1, AP-2, NF-κB, and HIF- 1), as well as HSE sequences, metal response elements and stress-response elements. Therefore, the complex gene structure explains its high sensitivity to induction by diverse pro-oxidant and inflammatory stimuli including heme, dopamine, TNF-α, IL-1β, cysteamine, β-amyloid, H2O2, hyperoxia, UV light, heavy metals, lipopolysaccharide, etc. [144]. In vertebrates HO-1 is shown to be upregulated by its substrate heme as well as by a wide variety of stressors including heavy metals, heat shock, ischemia, ROS, RNS, bacterial endotoxins, radiation, hypoxia, H2O2, nitric oxide, etc. [145-146]. Furthermore, inflammatory mediators such IL-1, TNF-α, LPS are also shown to upregulate HO-1 in vitro [147- 148]. At the cellular level, HO-1 is highly expressed in the organs participating in degrading senescent red blood cells, including spleen, reticuloendothelial cells of the liver and bone marrow [149] as well as in macrophages [150] and dendritic cells [151]. In fact, HO-1 upregulation in various cells is shown to attenuate the expression of various proinflammatory genes [152-153]. Furthermore, HO-1 is of great importance for building immunocompetence. Indeed, induction of HO-1 in dendritic cells alters their maturation state and interaction with other cells [151, 154], including T lymphocytes [155-156] and macrophages [140, 146, 157-158].
Regulation of HO-1 activity as an adaptive response to stress is mediated via several key initiator and feedback control processes. In particular, the transcriptional regulation of the HO-1 gene is shown to be attributed to several transcription factors including Nrf2, Bach1[159-160], HIF-1 [161] and PPARs [162]. It seems likely that MAPK signaling is involved in HO-1 induction [163]. In particular, the anti-inflammatory cytokine IL-10 was shown to induce HO-1 expression via a p38 MAPKdependent pathway [152]. Indeed, the antiapoptotic effect of CO was shown to be mediated by the activation of the p38 MAPK signal transduction pathway and required the activation of the transcription factor NF-κB [164]. Furthermore, the phosphatidylinositol-3 kinase (PI3K)/Akt signaling also modulates HO-1 activity [165]. In addition, HO-1 is involved in suppression of the expression of the pro-inflammatory cytokine TNF-α, while an HO-1 inhibitor (zinc protoporphyrin) attenuated this effect [152]. Furthermore, HO-1 is an important regulator of cellular metabolism, and its activity may affect NADPH- and oxygen-consuming pathways, including fatty acid synthesis, oxidative metabolism of cytochrome p450, or modulation of ROS generation in phagocytes [140].
3.6 Chicken HO-1
Data on HO-1 expression and its protective actions in poultry production are very limited. In early 1990th , HO-1 was purified from liver microsomes of chicks pretreated with cadmium chloride [166]. The molecular weight of the enzyme was shown to be 33,000 Da and the pH optimum of the reaction was 7.4. It was also shown that Hg2+ inhibited HO-1 activity by 67% at 10 μM and totally at 15 µM. Comparison of sequences to those derived from cDNA sequences for the major inducible rat and human HO-1 showed 69% and 76% similarities, respectively [166]. Next year, a cDNA from a chick liver library that encodes for HO-1 has been cloned and sequenced [167]. The protein corresponding to this fragment of DNA was found to compose of 296 amino acid residues and has a molecular mass of 33,509 Da. The similarity of chick HO-1 to rat and human HO-1 (nucleotides 66% and amino acids 62%) was confirmed to be moderately high. It was also shown that Cd-dependent induction of HO-1 was due to increased transcription of the gene or stabilization of its message [167]. Similar to mammalian HO-1, chicken HO-1 has five exons and four introns [168]. In the DNA sequence there are consensus sequences corresponding to numerous transcription factor recognition elements, including AP-1, AP-2, NF-kB, C/EBP, c-Myc and a metal-responding element identified in the promoter region [168]. Furthermore, chick HO-1 promoter region responded to sodium arsenite, H2O2 and transition metals, but not to heme. The chick HO-1 promoter region also contains a unique sequence that localized at -3.7 kb upstream of the transcription start site of the chick HO-1 gene and subserves up-regulation of the gene by metalloporphyrins [169-170]. Furthermore, the chick HO-1 promoter region was shown to contain "expanded" by three base pairs AP-1 sites that are important for up-regulation of the gene by heme and cobalt protoporphyrin, but not other metalloporphyrins [170].
HO-1 could be detected in microsomes from all chick or rat organs studied, including spleen, testis and brain [171]. The effects of heme on the induction of mRNA and protein synthesis for HO-1 have been studied in primary cultures of chick embryo liver cells [172]. It was shown that heme increased (up to 20-fold) the amount of mRNA and the rate of HO-1 gene transcription in a dose-dependent fashion. In fact, 7-15 h after heme addition, the half-life of HO-1 mRNA was 3.5 h in the presence or absence of actinomycin D, while the half-life of heme-induced HO-1 protein was 15 h [172]. Similarities were observed with respect to regulation of HO-1 expression in primary chick embryo hepatocytes and chicken hepatoma cells [173]. It seems likely that HO-1 synthesis is under hormonal control. For example, the effects of various hormones on the induction of HO-1 in monolayer cultures in chick embryo hepatocytes were examined [174]. Indeed, insulin is shown to suppress the activity of basal as well as Co2+ -induced HO-1, while hydrocortisone suppressed the basal enzyme activity and slightly enhanced Co2+ -induced enzyme activity. In contrast, triiodothyronine caused a slight increase of both uninduced and induced levels of the enzyme [174].
There is a range of in vitro studies, mainly with embryonic chick cells, to address possible mechanisms of HO-1 induction by various metals. For example, in primary cultures of embryonic chick liver cells HO-1 activity was shown to be upregulated by inorganic cobalt [175]. Treatment of isolated chick embryo liver cells in vitro with sodium arsenite or melarsoprol also showed a potent induction of HO-1 [176]. In monolayer cultures of chick embryo liver cells the most potent HO-1 inducing action was exhibited by Co2+, Cd2+, Sb3+, As3+, and Au1+ followed by lower induction observed with Cu2+, Fe2+, and Fe3+ [177]. In contrast, adding Zn2+ (20 μM), Mn2+ (50 μM) or cysteine (400 μM) to Co2+ -treated cells blocked/inhibited the HO-1 induction. It seems likely that increased HO-1 activity by metal treatment is dependent on fresh RNA and protein synthesis since cycloheximide and actinomycin D blocked the induction of HO-1 [177]. The activity of HO-1in chick embryo is shown to be enhanced by cadmium chloride treatment [178]. It has been suggested that induction of HO-1 by drugs and metals occurs by different mechanisms. For example, a drug phenobarbitone induced HO-1 by increasing hepatic haem formation, while increases in HO activity by metals (cobalt, cadmium or iron) were not dependent on increased haem synthesis and were not inhibited by 4,6- dioxoheptanoic acid [179]. In cultured chick embryo liver cells, synergistic induction of HO-1 by iron, added with the phenobarbital-like drug, glutethimide was hemedependent [180]. Addition of an inhibitor of heme biosynthesis abolished the synergistic induction of heme oxygenase providing evidence for the heme-dependent mechanism of induction. Both HO-1 mRNA and protein levels were shown to correlate with changes in HO-1 activity indicating that glutethimide and iron induce HO-1 at the transcriptional level. Induction of the HO-1 gene by heme is shown to be fundamentally different from that produced by transition metals or sodium arsenite and expression of the HO-1 gene is highly conserved across species [181]. Notably, in chick embryo liver cell cultures, HO-1 responded to sodium arsenite treatment in a dose-dependent fashion, and the response was rapid and transient. Although 2.5 μM arsenite is shown to induce HO-1 four- to six-fold, this had no effect on degradation of exogenous heme [182].
It seems likely that similar to mammals, in birds HO-1 induction in stress conditions is mediated by various signaling pathways. For example, in chicken hepatoma cells, MAP kinases ERK and p38 are shown to be involved in the induction of HO-1, and at least one AP-1 element is involved in this response [183]. In particular, it was shown that the phenylarsine oxide (PAO), an inhibitor of protein tyrosine phosphatases, upregulated HO-1 gene activity in dose- and time-dependent fashion and both an AP-1 element and a metal responsive element were involved in the PAO-mediated induction of the HO- 1 activity [184]. Indeed, a short (1-15 min) exposure of normal hepatocytes to low concentrations (0.5-3 μM) of PAO is shown to produce a marked increase in mRNA and protein of HO-1, which occurs without producing changes in cellular glutathione levels or stabilization of HO-1 message [185]. Furthermore, preincubation of cells with inhibitors of protein synthesis decreased the ability of PAO to increase levels of HO-1 mRNA, suggesting that the inductive effect requires de novo protein synthesis. Addition of thiol donors abrogated the PAOmediated induction of HO-1 in a dose-dependent fashion. Addition of genistein, a tyrosine kinase inhibitor, blunted the induction produced by both PAO and heme [185]. It was shown that induction of the chicken HO-1 gene by sodium arsenite or cobalt chloride is mediated through oxidative stress pathway(s) by activation of AP-1 proteins [186]. It seems likely that vascular endothelial growth factor upregulates HO-1 protein expression in vivo in chicken embryo chorioallantoic membranes by a mechanism dependent on an increase in cytosolic calcium levels and activation of protein kinase C [187].
In chick embryo hepatocytes heme breakdown occurred predominantly, if not solely, by heme oxygenase [188]. It seems likely, that increased HO-1 expression in chicken embryos between internal (day 19) and external pipping (day 20) [189] is an adaptive mechanism responsible for increased protection of tissues during this stressful period of the ontogenesis. Similarly, increased concentrations of vitamin E and carotenoids were observed in chicken embryonic tissues at the same period of time [63], providing an effective protection at hatching. It is well known, that various phytochemicals can affect HO-1 activity [190-191], however, more research is needed to understand molecular mechanisms of their interactions. For example, sulpharaphane containing broccoli extract and four different essential oils were tested in the 2 week old broilers as feed additives for 3 weeks. The phytogenic feed additives increased HO-1 activity in the jejunum, but decreased it in the liver [192]. It is interesting to note that relative mRNA expression of HIF-1 (heart) was increased and HO-1 (heart and liver) was decreased at week 4 in broilers fed with high ME and protein diet [193]. From the aforementioned data it is clear that HO-1 is well investigated in avian species, however, its response to different stresses in commercial and wild birds are still not fully characterised.
Thus, an analysis of the published data leads to the conclusion that HSPs play a significant role in cell/organism protection against various stresses being an integral part of the vitagene network responsible for proteostasis maintenance.
4. Practical applications of HSP expression in poultry production
4.1 Heat stress and HSPs in avian species
The university of the HSR and conservation of proteins induced by heat stress were shown in experiments with various species. As mentioned above, studies on effects of heat stress on the expression of HSPs in avian species started in early 1980th [49-51]. Similarly, exposure of chick myotube cultures to an increased temperature (45°C) caused extensive synthesis of three major HSPs (25 kDa, 65 kDa and 81 kDa). When experimental cells were allowed to recover from heatshock treatment at 37°C for 6-8 h, HSP synthesis declined to levels comparable to those in control cultures maintained at 37°C [194-195]. Therefore, four major chicken stress mRNAs coding HSPs with apparent molecular weights of 88 kDa, 71 kDa, 35 kDa and 23 kDa were separated and their properties were studied [196]. Exposure of the 11-day embryonic chicken lens to elevated temperature (45°C) dramatically increased the synthesis of three HSPs with subunit molecular weights of 89,000, 70,000 and 24,000 Da. Furthermore, the functional half-lives at 37°C of the mRNAs encoding the lens HSPs were about 3-5 hr [197].
The intracellular distributions of the major heat shock proteins, HSP89, HSP70, and HSP24 were studied in chicken embryo fibroblasts stressed by heat shock, allowed to recover and then restressed [198]. It was shown that HSP89 was localized primarily to the cytoplasm and during the restress a portion of this protein was associated with the nuclear region. In contrast, significant amount of HSP70 was shown to move to the nucleus during stress. In general, the nuclear HSPs reappeared in the cytoplasm in cells allowed to recover at normal temperatures. It is interesting to note that sodium arsenite also induces HSPs and their distributions were similar to that observed after heat shock, except for HSP89, which remained cytoplasmic [198]. Reticulocytes, purified from the blood of quail and chickens, responded to heat shock by the synthesis of HSP90, HSP70 and HSP26 (quail) or HSP24 (chicken) and the depressed synthesis of many other proteins normally produced at a physiological temperature [199]. It was shown that the expression of each protein depended upon the particular temperature and duration of heat exposure. It was noted that HSP70 was constitutively synthesized and selectively partitioned between cellular compartments. Furthermore, heat shock induced synthesis of the HSP90, HSP70 and HSP26 in quail was prevented by actinomycin D [199].
Heat shock response is a universal biological protective mechanism in stress conditions. Indeed, cultured bovine, equine, ovine and chicken lymphocytes responded to heat stress by the increased synthesis of HSPs. In particular, HSP70 and HSP90 were synthesized in all species and induction time of the HSPs synthesis comprised 30-60 minutes [200]. Heat shock response is an important mechanism of immune cells protection. Actually, heat-induced chicken macrophages synthesized HSP23, HSP70 and HSP90. The optimal temperature and time for induction of these HSPs was 45-46°C for 1 h, with a variable recovery period for each HSP [201]. A comparison of HSP synthesis among peritoneal macrophages (PM) from chickens, turkeys, quail, and ducks shows the highly conserved nature of heat-shock response within birds. In fact, macrophage cultures from each avian species expressed the three major HSPs (HSP23, HSP70 and HSP90) following heat-shock exposure (1-h heat shock at 45°C) [202]. There was also increased expression of a new HSP called P32, which probably was HSP32 (known as HO-1) in all 4 species. The authors also showed that the duck P32 and HSP23 were lower in molecular mass than their respective homologues expressed in chickens, turkeys, and quail macrophage cultures indicating some species-specific differences between HSPs in avian species [202]. Chicken macrophages (mononuclear phagocytic cell line MQNCSU) exposed to LPS under control (41°C) temperatures expressed enhanced synthesis of classical HSP23, HSP70, and HSP90, as well as heat-inducible 32- kDa protein (P32), and a novel LPS-induced 120-kDa protein (P120). In comparison to LPS treatment, MQNCSU cells exposed to 45°C (HS) expressed HSP23, HSP70, HSP90, and P32 but not P120 [203]. It is interesting to note that lead acetate caused a similar upregulation of the same four HSPs (HSP23, HSP70, HSP90 and P32) previously expressed by macrophages after in vitro and in vivo heat treatment [204]. It seems likely that various nutritional deficiencies could affect HSP response. For example, during acute in vivo heat stress, a HSP response was not inducible in chickens deficient in inorganic phosphorus [205] and they were more susceptible heat stress.
Increased HSPs expression in response to various stresses, including heat stress, is shown to be a universal mechanism in various chicken tissues. For example, both the amount and polyadenylation of HSP70 and ubiquitin transcripts increased when male germ cells from adult chicken testes were exposed to elevated (46°C) temperatures [206]. Similarly, there was a marked increase in HSP70 expression in the brains of female broiler chickens after 4 days (from d35 to d38) of heat treatment (38+/-1 degrees C for 2 h/d) [207]. In addition, in chicken pineal cells several heat shock proteins (HSPs 25, 70, and 90) are shown to be synthesized under temperature conditions [208]. Thermal stress (41°C) caused induction of HSP90α and HSP90β in chicken heart, liver and spleen, but HSP90α and HSP90? mRNA levels were stable in brain. Transcription of HSP70 also increased in all organs from chickens in heat stress groups when compared to chickens in control groups [209]. The elevation of the three HSPs in heart, may act as protective mechanism in adverse environments. For example, three main chicken HSPs (HSP60, HSP70, HSP90), and their corresponding mRNAs in the heart tissue of heat-stressed (37°C for 2-10 hours) broilers, elevated significantly after 2 h of heat exposure and decreased quickly with continued heat stress. However, the level of HSP60 protein in the heart increased and maintained throughout heat exposure [67].
Indeed, there is a great diversity in heat shock response in different tissues. For example, thirty two week old broiler breeders were subjected either to acute (step-wisely increasing temperature from 21 to 35°C within 24 hours) or chronic (32°C for 8 weeks) high temperature exposure. There was a tissue specificity in the response to acute and chronic stress [210]. For example, in the heart, acute heat challenge increased lipid peroxidation and upregulated gene expression of all four HSFs. Furthermore, during chronic heat treatment, the HSP70 mRNA level was increased and HSP90 mRNA was decreased. At the same time, in the liver, protein oxidation was alleviated during acute heat challenge and gene expression of HSF2, 3 and 4 and HSP70 were highly induced. In addition, HSP90 expression was increased by chronic thermal treatment. In the muscle, both types of heat stress increased protein oxidation, but HSF and HSP gene expression remained unaltered and only tendencies to increase were observed in HSP70 and HSP90 gene expression after acute heat stress [210]. The expression of HSP27, HSP70, and HSP90 mRNA in the bursa of Fabricius and spleen of 42-d old chickens were increased due to heat stress (37 ± 2°C for 15 d). However, under the same stress conditions the expression of HSP27 and HSP90 mRNA in the thymus was decreased. In the testes of heat-stressed cockerels (38 °C for 4 hours) the heat shock proteins, chaperonin containing t-complex, and proteasome subunits were downregulated [211]. Therefore, acute heat stress impairs the processes of translation, protein folding, and protein degradation resulting in apoptosis and spermatogenesis disturbance. Heat stress in 21-day-old broilers was associated with upregulation of the rectal temperature and the mRNA expression of HSP70 in the liver [212]. Heat stress (40°C for 2 h) in the growing chickens (41 day old) caused significant increases in sera corticosterone, LDH, MDA and SOD, the expression of HSP90 and HSP70 in the pectoralis major. Furthermore, HSP90 was shown to positively correlate with corticosterone and SOD activities [213]. In the chicken hypothalamus the transcripts of HSP90 decreased while HSP40 increased in response to thermal stress (34°C for 24 h) [214].
It seems likely that gene expression changes due to heat stress are of great importance for cell adaptation to stress. For example, heat stress (38°C for 4 hours) was associated with upregulation of 169 genes and downregulation of 140 genes in rooster testes [215]. Differentially expressed genes were mainly related to response to stress, transport, signal transduction, and metabolism. Indeed, HSP genes (HSP25, HSP70 and HSP90AA1) and related chaperones were the major upregulated groups in chicken testes after acute heat stress. Heat stress in chickens was associated with 166 differentially expressed genes in the brain, 219 in the leg muscle and 317 in the liver [216]. Six of these genes were differentially expressed in all three tissues and included heat shock protein genes (HSPH1-heat shock 105kDa/110kDa protein 1 and HSP25), apoptosis-related genes (RB1CC1, BAG3), a cell proliferation and differentiation-related gene and the hunger and energy metabolism related gene. Various functional clusters were related to the effects of heat stress, including those for cytoskeleton, extracellular space, ion binding and energy metabolism [216]. Terefore, it is proven that HSP expression in response to increased temperature is a universal cellular mechanism protecting proteins against unfavourable changes, including misfolding and molecular mechanisms of HSR need further research.
4.2 Thermal manipulation and HSPs
It has been noted that exposure of cells to nonlethal elevations in temperature activates cellular stress responses and induces a state of thermotolerance, characterised by increased cell resistance to subsequent lethal insults. Indeed, preconditioning is a phenomenon in which a prior stress provides protection against a subsequent and more severe stressful exposure/dose [217- 218], probably, via hermetic mechanisms. In fact, in early 1980th the idea of thermotolerance received substantial attention. In particular, in studies with Chinese hamster ovary cells thermal tolerance was shown to be developed during chronic or acute heating. For example, cells that expressed thermal tolerance as a result of a chronic heat treatment at 42°C also expressed thermal tolerance to a subsequent acute treatment at 45.5°C [219]. Also, cells heated acutely showed tolerance to chronic hyperthermia. Therefore, when cells are tolerant to chronic hyperthermia they are also tolerant to acute hyperthermia and the reverse is also true.
Physiological studies with wild birds and observations on the natural incubation of eggs by domestic hens indicate that egg incubation temperature has a great fluctuation [220] and it was hypothesized that this could have a positive effect on adaptive ability of chickens [221-222]. Therefore, exposing embryos to periods of high or low temperature during incubation could potentially affect their thermotolerance reflecting the programming effect of early development on the subsequent performance of chicken. Indeed, increasing the incubation temperature during important stages of the embryo development, associated with thermoregulation and stress, was shown to be an effective and long-lasting means of acquiring thermotolerance in growing chickens reared in cages [223-224]. Similarly, exposure of chicken embryo to a high incubation temperature (39.5 °C for 12 h/d between E7 to E16) reduced abdominal fat pad by 8% and increased breast muscle yield [225], and improved FCR [226]. Recently it has been shown that an increasing incubation temperature during early embryogenesis positively influences the growth and carcass traits of the broilers, accompanied with a partly negative impact on meat quality (drip loss, shear force, lightness) [227]. It is interesting to note that the growth effects were sexdependent, as significant weight differences could be only found in female broilers. Furthermore, it was concluded that thermal manipulation (TM) during turkey embryogenesis might have altered the thermoregulatory set point, and thus lowered the embryo metabolic rate with a long-lasting posthatch effects [228]. In fact, during the first week posthatch, myoblast proliferation activity was significantly higher in TM groups compared to controls; however, at 2 wk of age it was significantly lower [229]. Therefore, TM of the chick embryo has been suggested to improve the ability of the chicks to reduce their heat production during thermal stress later in their life. Furthermore, TM was shown to affect embryo physiology, growth, meat yield and processing quality [ 230-234] as well as heat tolerance, associated with lower body temperature until slaughter age [223-224].
However, the data on the effect of TM on thermotolerance published to date are not consistent and several studies reported no effect. Indeed, short periods 1 or 2 °C changes in incubation temperature did not influence hatching efficiency of broilers [231-232, 234- 237] or laying chicks [238]. For example, a higher egg shell temperature within the first embryonic week increases the weight of 21.5 d old broiler embryos but not of 7 d old chicks [239]. Similarly, incubation at higher temperatures between ED 7 and 10 resulted in comparable weights of broilers at day 36 post-hatch [240]. Lower carcass yields of 33 d old broilers and lower live weights and leg weights of 69 d old birds was reported after increasing incubation temperature (+1?C) between ED 3 and 6 compared to embryos at the normal incubation temperature [241]. In general, there was no negative effect of higher or lower temperatures from day 10 to 18 of incubation on hatching performance and hatch weight of laying chicks and prenatal temperature conditioning of laying hen embryos had no advantage on laying performance of hens under temperature stress conditions [242]. Principally, a critical time window of thermal manipulation of the chicken embryo is quite narrow since eggshell temperature manipulations (38.4- to 39.0?C for three hours daily) applied during hatching term (days 19 to 21) negatively affected incubation results and broiler performance, especially mortality due to ascites [243].
It seem likely that inconsistency of the results of TM during avian embryonic development reflects lack of understanding molecular mechanisms of the acquisition of TM. Therefore, even small variations in experimental set up (breed, sex, temperature as well as time and duration of its application, etc.) could substantially affect experimental results. There is no doubt that TM during embryogenesis has long-lasting effects on physiology, which may potentially modulate gene expression and metabolism in peripheral tissues being a background for an adaptive mechanism such as heat tolerance [225]. Without affecting hatchability, TM resulted in lower body temperature at hatching and until d 28 post-hatch and significant changes in plasma thyroid hormone concentrations. Notably, fine tuning of incubation conditions, taking into account the level and duration of increases in temperature and relative humidity during a critical period of embryogenesis is considered to be a powerful tool for improvement thermotolerance (resistance to environmental changes) and growth and development of the posthatch chicks [244]. It seems likely that the induction of epigenetic mechanisms related to control body temperature provides long lasting effect in postnatal development of birds. In fact, DNA methylation and histone modification patterns during the late embryonic and early postnatal development of chickens have been recently described [245] and it would be important to study effects of TM on these epigeneticrelated processes.
Recently the effects of increasing the incubation temperature by 1 °C from day 11 to 20 on the embryonic and posthatch skeletal muscle development of the Peking duck were investigated and gene expression profile of leg muscle tissues from thermally manipulated ducks was assessed [246]. Indeed, altering the incubation temperature had immediate and long-lasting effects on phenotypic changes in the embryonic and post-hatching muscle development. In particular, expression levels of total 1370 genes were altered in muscle tissues by the thermal treatments. In fact, cellular processes including metabolism, cell cycle, catalytic activity, and enzyme regulatory activity may have involved in the muscle mass impacted by thermal manipulation [246]. The transcriptome studies confirmed the complexity of the heat stress response. In fact, heat stress responsive genes in the chicken male white leghorn hepatocellular cell line have been identified [247]. The transcripts of 812 genes were shown to respond to heat stress with 235 genes upregulated and 577 downregulated following 2.5 h of heat stress. Genes whose products function as chaperones, as well as genes affecting collagen synthesis and deposition, transcription factors, chromatin remodelers, and genes modulating the WNT and TGF-β pathways were upregulated. At the same time, genes affecting DNA replication and repair along with chromosomal segregation were found to be predominant among the downregulated genes [247].
As mentioned above, molecular mechanisms of acquired thermotolerance are not fully understood but HSP expression and synthesis are thought to play an essential part in this process. Initial work on effect of early chick exposure to heat stress on the HSP expression and thermotolerance in later life started at the end of 1990th. In fact, when chicks at early age were exposed to heat (conditioning; 36°C for 24h at age of 5 days) to improve thermotolerance, lower levels of HSP induction in the heart and lung tissues was observed in the treated chickens [248]. It was shown that the induction of HSP in the heart and lung tissues of the whole animal correlates with the body temperature. Indeed, at the age of 42 days, challenge with acute heat stress (35°C) resulted in a large increase in cloacal temperature of the control chickens and by a more moderate increase in the conditioned chickens. Mortality during the thermal challenge was significantly higher in the control chickens than in the conditioned ones. However, the synthesis rate of HSP70 and HSP90 during the first hour of heat challenge, accelerated gradually in control chickens, whereas in the conditioned chickens it accelerated only after 3 hours and in a more moderate response [249]. The authors suggested that HSP response does not represent a part of the longterm mechanism that is evoked by the early age conditioning. However, next year that conclusion was challenged. In fact, heat conditioning (41°C for 1 h, daily) in both broiler chickens and turkey poults enhanced expression of HSP90, HSP70, and HSP23 in peripheral leukocytes when these cells were heat stressed in vitro [250]. In chickens, 1wk conditioning was sufficient to enhance the HS response when the leukocytes were heat stressed in vitro in the following week. However, in turkey a 3-wk heat conditioning period, followed by a 2- wk rest period, was associated with maximal induction of the three HSP studied in these experiments. It is interesting to note that enhancement in HSP expression was evident for periods up to 4 wk after termination of the daily heat conditioning episodes [250]. This was further developed in a study where resistance to acute heat stress (41°C, days 39-42) and concentration of HSP70 were increased in chickens subjected to early heat stress during rearing (3 heat stress episodes at 35°C for 4 h per week) [251]. Furthermore, a positive correlation was observed between HSP70 concentration and the time taken for a 3°C increase in rectal temperature. It was shown that a combination of early feed restriction (days 4-6) and heat stress (36°C for 1 hour from day 1 to day 21, FRHT) was associated with better HSP70 response after heat stress (d 41). Furthermore, early stress improved weight gain and resistance to IBD in male broiler chickens under heat stress conditions [252]. The authors attributed the improved heat tolerance and disease resistance in FRHT birds to better HSP 70 response.
Ten years later the interest in role of HSPs in chicken thermotolerance was renewed. TM during embryogenesis (39°C for 9-18h) resulted in a significant increase in mRNA levels of HSP90, HSP60 and HSF1 in muscle, heart and brain tissues during embryogenesis and during thermal challenge (43°C) at days 10 and 28 posthatching [253]. The authors suggested that the changes in HSP90, HSP60 and HSF1 gene expressions could be associated with improved thermotolerance acquisition in TM chicks. However, a recent research from Rajkumar et al. [254] showed that exposure to increased temperature (by 2°C) during incubation (days 16-18) resulted in reduced expressions of HSP70 mRNA in various tissues indicating better thermotolerance of the heat-exposed birds. It seems likely that temperature, duration of heat treatment, stages of embryo development and other differences in expe-rimental set up could be responsible for a variability in HSP response.
It seems likely that long-term results of TM of the avian embryo and thermotolerance acquisition are mediated not only by HSPs, but other molecular mechanisms are also involved. For example, the role of HSP in acquisition of thermotolerance was questioned, since a major inducible HSP, HSP68, was not required for the development of thermotolerance in rat fibroblasts [255] or mouse plasma cytoma cells [256]. Furthermore, it was demonstrated that P388D1, a mouse macrophage tumour cell line, failed to induce HSPs in response to either heat stress (42°C, 1 h) or to heavy metal stress induced by arsenic trioxide (5–20 µM), however, cells exhibited thermotolerance in the absence of induced HSPs. The tolerance was shown to be abrogated in cells treated with cycloheximide (250 ng/ml) suggested that thermotolerance was dependent on de novo protein synthesis [257]. Furthermore, posttranslational histone modifications in the promoters of HSP80 and HSP90 are suggested to be involved in formation of heatacclimation-mediated cytoprotective memory [258]. Indeed, there is a need for more research in this area to elucidate molecular mechanisms of thermotolerance with a specific emphasis to HSPs and their interactions with various elements of the antioxidant defence system, including transcription factors.
4.3 Heavy metals and HSPs
Heat shock proteins are important cellular tools in protection against heavy metal toxicity. For example, splenocytes harvested in presence of sodium arsenite were characterised by increased expression of HSP70 and serum levels of HSP70 in broiler chicken also increased after continuous supplying sodium arsenite in drinking water [259]. Similarly, the levels of HSP mRNA (HSP90, HSP70, HSP60, HSP40 and HSP27) and protein (HSP70 and HSPp60) expression in immune organs of chickens were significantly increased in the As2O3 treatment groups compared with the corresponding control groups [260]. However, HSP response depends on the toxicant dose used. For example, addition of lead to the chicken diet (200 mg lead acetate/kg diet) significantly decreased feed intake, body weight gain, and feed efficiency, upregulated the antioxidant enzymes gene expression together with the downregulation of glutathione Stransferase and HSP70 in the jejunum [261]. In an in vitro study, Mn had a dosage-dependent effect on HSP27, HSP40, HSP60, HSP70, and HSP90 mRNA expression in chicken spleen lymphocytes: the mRNA expression of the heat shock proteins was induced at lower concentrations of manganese and was inhibited at higher concentrations [262]. Therefore, as manganese concentration increased, the mRNA expression of the heat shock proteins first increased and then decreased.
4.4 Dietary AO and HSPs
Since all antioxidants in the body are working together to build the effective antioxidant defence network, the increased concentration of one antioxidant can be associated with down-regulation of another antioxidant element in stress conditions.
4.4.1 Vitamin E
Vitamin E is considered to be a main chainbreaking antioxidant in biological systems and its roles in poultry production are greately appreciated [3, 63, 263- 264Ç. It was shown that vitamin E, added to the Vero cell culture prior mycotoxins (citrinin, zearalenone and T2 toxin) was able to prevent an induction of HSP70 expression due to mycotoxins [265]. In isolation-stressed quail, vitamin E or vitamin C were shown to prevent an increase in HSP70 expression in the brain and heart [266]. In crossbred cows, treatment with α-tocopherol acetate during dry period resulted in reduced oxidative stress and HSP70 [267]. In cultured rat hepatocytes vitamin E significantly counteracted the effect of cyclosporine Ainduced increase in HSP70 [268]. Furthermore, in young men, α-tocopherol was shown to prevent the exercise induced increase of HSP72 in skeletal muscle as well as in the circulation [269]. However, in most of cases effect of vitamin E on HO-1 expression is different from the aforementioned effects on HSP70. Indeed, recently it has been shown that vitamin E activated the HO-1 promoter via the cAMP-response element, but not the ARE enhancer, through the extracellular signal-regulated kinase and protein kinase A [270]. It was shown that α-Tocopheryl succinate (α-TOS) increases nuclear translocation and electrophile-responsive/antioxidantresponsive elements binding activity of Nrf2, resulting in up-regulation of downstream genes cystine-glutamic acid exchange transporter and HO-1, while decreasing NF-κB nuclear translocation [271]. It seems likely that α- tocopherol protects human retinal pigment epithelial cells from acrolein-induced cellular toxicity, not only as a chain-breaking antioxidant, but also as a Phase II enzyme inducer, including Nrf-2, SOD and HO-1 induction [272]. Similarly, in a murine prostate cancer model γ-tocopherol-enriched mixed tocopherols significantly upregulated the expression of Nrf2 and its related detoxifying and antioxidant enzymes, including SOD and HO-1 [273]. In rats, protective effect of vitamin E against focal brain ischemia and neuronal death was shown. In fact, vitamin E induced the expression of the alpha subunit of hypoxia-inducible factor-1 (HIF-1) and its target genes, including vascular endothelial growth factor (VEGF) and heme oxygenase-1 [274].
4.4.2 Ascorbic acid
Ascorbic acid is the most important water-soluble antioxidant provided with feed and synthesised within the animal/chicken body [275]. It has been shown that chickens experience a less severe stress response after exposure to high temperatures when they are provided dietary ascorbic acid. In fact, heart HSP70 expression decreased in ascorbic acid-fed chickens and the HSP70 increase after heat was two-fold lower in ascorbic acidfed birds in comparison with the control chickens. Furthermore, plasma corticosterone and heart HSP70 were positively correlated, while plasma ascorbic acid and heart HSP70 were negatively correlated [276]. In the ascorbic acid-fed chickens, neither the lower constitutive HSC70 nor the decreased HSP70 response to heat stress (42°C) in the heart and liver were sex-dependent [277]. A lower expression of HSP70 associated with lower body temperature in heat-stress conditions reflected a lower stress response in the ascorbic acid-fed birds. Indeed, ovary and brain HSP70 expression linearly decreased as dietary vitamin C or vitamin E supplementation increased in heat-stressed quail. However, HSP70 expression of ovary and brain was not affected by vitamin C or E supplementation under thermo-neutral conditions [278].
Effects of ascorbic acid on HSP70 expression were also evaluated in experiments with laboratory animals or in human trials. For example, lymphocytes from nonsupplemented subjects responded to hydrogen peroxide with increased HSP60 and HSP70 content over 48 h. In fact, in vitamin C supplemented subjects, baseline HSP60 (lymphocytes) and HSP70 (muscles) content were elevated, but they did not respond to hydrogen peroxide or exercise [279]. In elderly, increased concentration of vitamins C and E was associated with a reduction in oxidative stress and leukocytes HSP72 [280]. Ascorbic acid was shown to attenuate increase in HSP expression due to various toxic agents or heat stress. For example, human brain astrocyte cells enriched with ascorbic acid before being exposed to ethanol, were reported to be better protected against the alcohol-mediated toxicity than non-supplemented cells, and showed significantly lower concentrations of HSP70 [281]. Ascorbic acid significantly attenuated Cd-induced upregulation of GRP78 in mouse testes [282]. ?yclic heat stress (23 to 38 to 23°C, for 2 h on each of seven consecutive days) activated hepatic HSP70, TNF-α, iNOS, and GSH-Px genes, whereas vitamin C (0.5% in water) during heat stress ameliorated heat stress-induced cellular responses in rats [283]. It is interesting to note that there was a specific disappearance of HSP70 in the testes of 20-dayold ascorbic acid-deficient mice [284]. It seems likely that effects of ascorbic acid on HSPs is not universal and for HO-1 is different from HSP70. Indeed, the HO-1 mRNA and protein level in rat kidney, liver, and lung were highly induced by ascorbate treatment (100 mg/kg b.w.) under normal and HS conditions. In particular, in HS the HO-1 activity in tissues was enhanced by both ascorbate preand post-treatment [285].
4.4.3 Vitamin D3
Vitamin D is known for its classical functions in calcium uptake and bone metabolism. However, recently, vitamin D has been recognized for its non-classical actions including modulation of antioxidant defences [286-287] through regulating oxidant and antioxidant enzyme genes. It was shown that HO-1 was downregulated in the livers of mice fed the vitamin D deficient diet [288]. At the same time, vitamin D deficiency increases the expression of the hepatic mRNA levels of HO-1 in obese rats [289]. In a model of reperfusion of bilateral femoral vessels pre-treatment of rats with vitamin D3 results in a significant increase in leukocyte HO-1 expression in rat model of reperfusion [290]. By employing microarray technology, the effect of a single dose of 1,25-(OH)2D3 on gene expression in the intestine of vitamin D-deficient rats was evaluated. Indeed, at 3 h, there was a 1.9-fold increased expression of HO-1 [291]. The effects of 1,25-D3 treatment on HO-1 expression following focal cortical ischemia elicited by photothrombosis in glial cells were studied. Postlesional treatment with 1,25-D3 (4 μg/kg body weight) resulted in a transient, but significant upregulation of glial HO-1 immunoreactivity [292].
4.4.4 Carnitine and betaine
Carnitine is considered as a novel mitochondriatargeted antioxidant with a range of antioxidant actions [1], while betaine is reported to have antioxidant properties in various oxidative stress-generating model systems [293]. In human endothelial cells in culture carnitine was shown to increase gene and protein expression of HO-1 [294]. Furthermore, in humans and in an animal model it was shown that carnitine-mediated improved response to erythropoietin involves induction of HO-1 [295]. Indeed, L-carnitine treatment was associated with an increased level of HO-1 in the retinal ganglion cells [296]. L-Carnitine prevented increase in HSP70 in the testes of cadmium-exposed rats [297]. It was shown that Acetyl-L-carnitine-induced up-regulation of heat shock proteins protects cortical neurons against amyloidbeta peptide 1-42-mediated oxidative stress and neurotoxicity [298]. Acetylcarnitine induces heme oxygenase (increased the amount and activity of HO) in rat astrocytes and protects against oxidative stress [299]. From the aforementioned data it is clear that carnitine can be considered as an important regulator of the vitagene network.
The influence of hyperosmotic shrinkage and the osmolyte betaine on heme oxygenase expression was studied in cultured rat hepatocytes. Hyperosmolarity transiently suppressed HO-1 induction in response to hemin or medium addition at the levels of mRNA and protein expression. Pretreatment of the cells with betaine largely restored induction of both HO-1 mRNA and protein under hyperosmotic conditions [300].
4.4.5 Selenium
Selenium is a central part of the antioxidant defence network via at least 25 selenoproteins [32]. The protective effect of selenium against cadmium-induced cytotoxicity in chicken splenic lymphocytes was shown to be mediated via the HSP pathway [301]. Indeed, the mRNA expression of HSPs (HSP27, HSP40, HSP60, HSP70 and HSP90) exposed to 10-6 mol/L Cd showed a sustained decrease at 12-48 h exposure. Notably, adding to the medium Se (10-7 mol/L) was associated with a significant increase in the mRNA expression of HSPs, as compared to the control group of chicken splenic lymphocytes. Concomitantly, treatment of chicken splenic lymphocytes with Se in combination with Cd prevented a decrease in the mRNA expression of HSPs due to Cd treatment. A different HSP response to arsenic was observed. The expression of HSPs mRNA and protein (HSP70 and HO-1) in rat liver were increased by 5- and 3-folds in the arsenic-fed animals compared with the control group, and selenium prevented the occurrence of oxidative damage from arsenic and significantly reduced expression of HSPs mRNA and protein [302].
The HSP70 response was shown to be significantly lower in the chickens fed selenium and challenged with either enteropathogenic Escherichia coli or heat stress than in those chickens given no supplemental selenium [303-304]. An acute heat stress induced HSP70 in 22d turkey embryos and the embryos from selenium-fed dams were shown to have less HSP70 after the 3 h post-heat stress recovery period [305] demonstrating that selenium had the ability to reduce the impact of heat stress. In fact, heat stress enhanced HSP70 and HSP27 expression and concentration in chicken spleen and dietary Se prevented the aforementioned increase in HSPs [306]. Similarly, in piglets under heat stress conditions selenium can downregulate the mRNA levels of HSPs in various tissues [307]. The relative messenger RNA (mRNA) and protein expression of HSP60, HSP70, and HSP90 in PBMC was observed highest in heat-stressed goats and Se + vitamin E supplementation decreased the HSP expression [308].
In contrast, Se deficiency increased the mRNA levels of HSPs (HSP90, 70, 60, 40, and 27) in chicken neutrophils [309]. Indeed, HSPs played an important role in the protection of the chicken liver after oxidative stress due to Se deficiency. For example, the mRNA levels of HSPs and the protein expression of HSPs (HSP60, 70, and 90) increased significantly in the Se-deficient group compare to the corresponding control group [310]. In exudative diathesis (ED) broiler chicken model caused by Se deficiency, the antioxidant function was shown to decline remarkably, and most of the HSP expression levels increased significantly in the spleen, thymus, and bursa of Fabricius of the broiler chicks with ED [311]. Indeed, Se deficiency causes defects in the chicken bursa of Fabricius associated with decreased selenoprotein expression [312]. As a compensatory response to changes due to Se deficiency, the mRNA and protein expression levels of HSPs (HSP27, HSP40, HSP60, HSP70, and HSP90) were significantly increased. Similar observations with Se deficient mouse were recorded. For example, Se deficiency was shown to increase HSP70 levels in mouse testes [313]. A significant increase in the stress-inducible HSP70 gene and protein expression was observed in the mice fed Se-deficient or Se-excess supplemented diet as compared with Se adequate fed group [314]. It is interesting to note that the testis-specific HSP70-2 expression significantly decreased as result of Se deficiency. It is clear that increased expression of HSPs in response to toxic metals is an adaptive mechanism to deal with oxidative stress imposed by such toxicants. Similarly, in the case of Se deficiency increased HSP expression is also an adaptive mechanism to compensate for lack of synthesis of selenoproteins and their antioxidant protective functions. As mentioned above, HSP response to various stressors and to nutritional supplements would depend on many factors, including the model used, stressor nature and strength, etc. For example, in human lens epithelial cells sodium selenite gradually increased the expression of HSP70 in a timedependent manner [315]. In rat hippocampus with ischemia-induced neuronal damage, selenium pretreatment was shown to significantly increase the level of HSP70 when compared with ischemic group [316]. In fact, a significant increase in hippocampal HSP70 expression in the ischemic group was observed but the expression was even higher in the selenium-pretreated group than ischemic group.
4.4.6 Phytochemicals
Regulatory and health promoting properties of various phytochemicals and their effects on HSPs have received substantial attention and there is a range of comprehensive reviews covering the subject [317-320]. They are beyond the scope of the present review. Therefore, only effects of silymarin, possessing a range of antioxidant-related activities [2], are reviewed below.
4.4.6.1 Silymarin
It seems likely that SM, similar to other flavonoids, can affect the vitagene network. In fact, SM/silybin affects HSP32 (HO-1) activity in different model systems. For example, As-intoxicated rats showed a significant upregulation of myocardial NADPH (NOX) oxidase subunits such as NOX2 and NOX4 as well as Keap1 and down-regulation of Nrf2 and vitagene HO-1 protein expressions. Pre-administration of silibinin (75 mg/kg/BW) recovered all these altered parameters to near normalcy in As-induced cardiotoxic rat [321]. Similarly, in a model of liver injury caused by alcohol plus pyrazole, SM administration (50 mg/kg/BW) had a protective effect with a trend in restoring the decreased activity of HO-1 and Nrf2 [322].
SM (250 mg/kg/BW) possesses substantial protective effect against B(a)P-induced damages by increasing (restoring) HO-1 (vitagene) activity [323]. Similarly, in vitro SM (500 μM) reduced tBH-induced hepatocyte toxicity by activating HO-1 gene expression [324]. Indeed, the enzyme HO-1 is an important regulatory molecule present in most mammalian cells. In fact, the main function of HO-1 is to break down the prooxidant molecule heme into three products; carbon monoxide (CO), biliverdin and free iron and actively participate in the antioxidant defence in the human/animal body [325]. Indeed, HO-1 is a stress-inducible protein and can be induced by various oxidative and inflammatory signals. From the data presented above it is clear that SM/silibinin can upregulate HO-1 and improve antioxidant defences. It is likely that SM/silibinin can affect other HSPs including HSP70. Indeed, in an in vitro system based on CHO-K1 cells treated with As, SM (5 μM) significantly decreased HSP70 expression previously elevated by As [326]. In another in vitro system based on heat-induced chicken hepatocytes, SM (259 μM) affected HSP70 expression significantly, preventing its alleviation by heat stress [327]. A similar protective effect of SM (100 mg/kg/BW) on HSP70 was seen in rats given SM for 7 days prior to mesenteric ischemia-reperfusion (I-R) compared to I-R group [328]. It is interesting to note that silybin was identified as a novel HSP90 inhibitor [329]. Therefore, silibinin can decrease HSP70 expression in stressed cells indicating improved AO defences and decrease stress by other means (e.g., Nrf2-related increased AO synthesis). Indeed, effects of silymarin on HSPs in avian species awaits investigation, while other phytochemicals are shown to be effective. For example, resveratrol, a plant phytochemical possessing antioxidant activities, attenuated the heat stress-induced overexpression of HSP27, HSP70, and HSP90 mRNA in the bursa of Fabricius and spleen and increased the low expression of HSP27 and HSP90 mRNA in thymus in 42 d old chickens upon heat stress [330]. Indeed, there is a need for more detailed investigation of the relationship between nutritional antioxidants and HSP expressions in physiological and stress conditions.
4.5 Nutritional modulation of vitagenes
The aforementioned data clearly indicate that vitagenes can be modulated by nutritional means. Indeed, Vitamins E, D, C, carnitine, betaine, selenium and some phytochemicals can affect HSP expression and concentration in various stress conditions. It is interesting that the same compounds can affect other vitagenes, namely thioredoxins, sirtuins and SOD [331]. Therefore, it would be of considerable interest to develop an antioxidant-based composition/supplement for decreasing negative consequences of various stresses in poultry and pig production. Such a composition should meet at least five important requirements [1]:
- Vitagene activation and redox-signaling (carnitine, betaine, vitamins A, E, D, C, Se, Zn, Mn, silymarin and possibly other phytochemicals);
- Maintenance of the vitamin E recycling system (vitamin C, Se, Vitamin B1 and B2);
- Provision of nutrients required for carnitine synthesis (lysine and methionine, ascorbic acid, vitamin B6 and niacin);
- Supporting the liver, a main site of T-2 toxin, ochratoxins, fumonisins and aflatoxins detoxification and gut, responsible to DON detoxification (vitamins E and C, selenium, carnitine, betaine, organic acids, methionine and lysine);
- Maintaining high immunocompetence (vitamins A, E, D, C, carnitine, Se, Zn and Mn).
Inclusion of various protective compounds into the diet of farm animals and poultry to decrease negative consequences of stress conditions is complicated, firstly, by a decreased feed consumption at time of stress. Secondly, such an approach has a low flexibility, since the existing feeding systems do not allow to include anything into the feed loaded into the feed storage bins located near the poultry/pig house (usually several tons of feed for several days feeding). Therefore, before the previous feed is consumed, nothing can be added to the feed. However, sometimes it is necessary to supplement animals/poultry with specific additives very quickly to deal with consequences of unexpected stresses (e.g. mycotoxins in the feed, immunosuppression, high temperature, etc.). In such a case, additive supplementation via drinking system is a valuable option [331]. In fact, modern commercial poultry and pig houses have water medication equipment installed, which can be perfectly used for the aforementioned supplementations. For example, an attempt to address the aforementioned option was implemented in a commercial product PerforMax, containing a vitagene-regulating mixture of 28 compounds, including antioxidants (vitamins E and C), carnitine, betaine, minerals (Zn and Mn) and essential amino acids, and supplied via drinking water. Its efficacy in fighting stresses in commercial poultry production has been recently reviewed [4] and prospects of its use to maintain gut health in weaned piglets and newly hatched chicks was considered [5].
Indeed, it is well known that commercial animal/poultry production is associated with a range of stress conditions including environmental (high temperature), nutritional (mycotoxins and oxidized fat) or internal (vaccinations, disease challenges, etc.) stresses [4, 32, 63]. In such conditions, supplying the PerforMax with drinking water was shown to have protective effects in growing birds [332-333] as well as in adult birds [4] helping maintain their health, productive and reproductive performance. Therefore, the aforementioned results are the first step to go from the development of the vitagene concept to designing a commercial product and testing it in the commercial conditions of poultry and pig production. We can suggest that this idea could be realized in human nutrition as well. Clearly more research is needed to understand a fundamental role of vitagenes in adaptation to various stresses.
5. CONCLUSIONS AND FUTURE DIRECTIONS
From the aforementioned analysis of the data related to HSPs in poultry physiology and adaptation to stresses it is possible to conclude:
- HSPs as important vitagenes are the main driving force in cell/body adaptation to various stress conditions. Indeed, in stress conditions synthesis of most cellular proteins decreases while HSP expression is usually significantly increased;
- HSPs being cellular chaperones are responsible for proteostasis and involved in protein quality control in the cell to prevent misfolding or to facilitate degradation, making sure that proteins are in optimal structure for their biological activities;
- There are tissue-specific differences in HSP expression which also depends on the strength of such stress-factors as heat, heavy metals, mycotoxins and other toxicants;
- HSP70, HSP90 and HSP32 are shown to be protective in heat stress, toxicity stress as well as in other oxidative-stress related conditions in poultry production;
- Molecular mechanisms of HSP participation in acquisition of thermotolerance need further detailed investigation;
- There are complex interactions inside the antioxidant systems of the cell/body to ensure an effective maintenance of homeostasis in stress conditions. Indeed, in many cases nutritional antioxidants (vitamin E, ascorbic acid, selenium) in the feed can decrease oxidative stress and as a result HSP expression could be decreased as well;
- Regulating effects of various phytochemicals on HSPs need further investigation;
- Protective effects of HSPs in the immune system in stress conditions await practical applications in poultry production;
- Nutritional means of additional HSP upregulation in stress conditions of poultry production and physiological and commercial consequences await investigation. Indeed, in medical sciences manipulation of HSP expression is considered as an important approach in disease prevention and treatment. It seems likely that in poultry/animal sciences nutritional manipulation of vitagenes is a new way in managing commerciallyrelevant stresses.
ACKNOWLEDGEMENT: None
CONFLICT OF INTEREST: The authors declare that they have no conflict of interest.
This article was originally published in Journal of Science / Vol 5 / Issue 12 / 2015 / 1188-1222.
6. REFERENCES
1. Surai PF. Antioxidant Action of Carnitine: Molecular Mechanisms and Practical Applications. EC Vet Sci, 2, 2015, 66- 84.
2. Surai PF. Silymarin as a Natural Antioxidant: An Overview of the Current Evidence and Perspectives. Antioxidants, 4, 2015, 204-247.
3. Surai PF. Polyphenol compounds in the chicken/animal diet: from the past to the future. J Anim Physiol Animal Nutr, 98, 2014, 19-31.
4. Shatskih E, Latipova E, Fisinin V, Denev S and Surai P. Molecular mechanisms and new strategies to fight stresses in egg-producing birds. Agric Sci Technol, 7, 2015, 3-10.
5. Surai PF and Fisinin VI. Antioxidant-Prooxidant Balance in the Intestine: Applications in Chick Placement and Pig Weaning. J Vet Sci Med, 3, 2015, 1-16.
6. Pockley AG and Multhoff G. Cell stress proteins in extracellular fluids: friend or foe? Novartis Foundation Symposium, 291, 2008, 86-95.
7. Velichko AK, Markova EN, Petrova NV, Razin SV and Kantidze OL. Mechanisms of heat shock response in mammals. Cell Mol Life Sci, 70(22), 2013, 4229-4241.
8. Meijering RA, Henning RH and Brundel BJ. Reviving the protein quality control system: therapeutic target for cardiac disease in the elderly. Trends Cardiovasc Med, 25(3), 2015, 243-247
9. Fujimoto M and Nakai A. The heat shock factor family and adaptation to proteotoxic stress. FEBS J, 277(20), 2010, 4112-4125.
10. Sakurai H and Enoki Y. Novel aspects of heat shock factors: DNA recognition, chromatin modulation and gene expression. FEBS J, 277(20), 2010, 4140-4149.
11. Takii R, Fujimoto M, Tan K, Takaki E, Hayashida N, Nakato R, Shirahige K and Nakai A. ATF1 modulates the heat shock response by regulating the stress-inducible heat shock factor 1 transcription complex. Mol Cell Biol, 35(1), 2015, 11-25.
12. Richter K, Haslbeck M and Buchner J. The heat shock response: life on the verge of death. Mol Cell, 40(2), 2010, 253- 266.
13. Liu Y and Chang A. Heat shock response relieves ER stress. EMBO J, 27(7), 2008, 1049-1059.
14. De Thonel A, Le Mouël A and Mezger V. Transcriptional regulation of small HSP-HSF1 and beyond. Int J Biochem Cell Biol, 44(10), 2012, 1593-1612.
15. Stetler RA, Gan Y, Zhang W, Liou A., Gao Y, Cao G and Chen J. Heat shock proteins: cellular and molecular mechanisms in the central nervous system. Prog Neurobiol, 92(2), 2010, 184-211.
16. Kantidze OL, Velichko AK and Razin SV. Heat stress-induced transcriptional repression. Biochemistry (Moscow), 80, 2015, 990-993.
17. Nakai A and Morimoto RI. Characterization of a novel chicken heat shock transcription factor, heat shock factor 3, suggests a new regulatory pathway. Mol Cell Biol, 13(4), 1993, 1983-1997.
18. Green M, Schuetz TJ, Sullivan EK and Kingston RE. A heat shock-responsive domain of human HSF1 that regulates transcription activation domain function. Mol Cell Biol, 15(6), 1995, 3354-3362.
19. Nakai A, Kawazoe Y, Tanabe M, Nagata K and Morimoto RI. The DNA-binding properties of two heat shock factors, HSF1 and HSF3, are induced in the avian erythroblast cell line HD6. Mol Cell Biol, 15(10), 1995, 5268-5278.
20. Tanabe M, Nakai A, Kawazoe Y and Nagata K. Different thresholds in the responses of two heat shock transcription factors, HSF1 and HSF3. J Biol Chem, 272(24), 1997, 15389-15395.
21. Tanabe M, Kawazoe Y, Takeda S, Morimoto RI, Nagata K and Nakai A. Disruption of the HSF3 gene results in the severe reduction of heat shock gene expression and loss of thermotolerance. EMBO J, 17(6), 1998, 1750-1758.
22. Kawazoe Y, Tanabe M, Sasai N, Nagata K and Nakai A. HSF3 is a major heat shock responsive factor during chicken embryonic development. Eur J Biochem, 265(2), 1999, 688-697.
23. Nakai A and Ishikawa T. A nuclear localization signal is essential for stress-induced dimer-to-trimer transition of heat shock transcription factor 3. J Biol Chem, 275(44), 2000, 34665-34671.
24. Inouye S, Katsuki K, Izu H, Fujimoto M, Sugahara K, Yamada S, Shinkai Y, Oka Y, Katoh Y and Nakai A. Activation of heat shock genes is not necessary for protection by heat shock transcription factor 1 against cell death due to a single exposure to high temperatures. Mol Cell Biol, 23(16), 2003, 5882-5895.
25. Nakai A and Ishikawa T. Cell cycle transition under stress conditions controlled by vertebrate heat shock factors. EMBO J, 20(11), 2001, 2885-2895.
26. Shabtay A and Arad Z. Reciprocal activation of HSF1 and HSF3 in brain and blood tissues: is redundancy developmentally related? Am J Physiol Regul Integr Comp Physiol, 291(3), 2006, R566-572.
27. Shinkawa T, Tan K, Fujimoto M, Hayashida N, Yamamoto K, Takaki E, Takii R, Prakasam R, Inouye S, Mezger V and Nakai A. Heat shock factor 2 is required for maintaining proteostasis against febrile-range thermal stress and polyglutamine aggregation. Mol Biol Cell, 22(19), 2011, 3571-3583.
28. Prakasam R, Fujimoto M, Takii R, Hayashida N, Takaki E, Tan K, Wu F, Inouye S and Nakai A. Chicken IL-6 is a heatshock gene. FEBS Lett, 587(21), 2013, 3541-3547.
29. Vihervaara A and Sistonen L. HSF1 at a glance. J Cell Sci, 127(Pt 2), 2014, 261-266.
30. Ritossa F. A new puffing pattern induced by temperature shock and DNP in drosophila. Cell Mol Life Sci, 18(12), 1962, 571–573.
31. Kalmar B and Greensmith L. Induction of heat shock proteins for protection against oxidative stress. Adv Drug Deliv Rev, 61(4), 2009, 310-318.
32. Surai PF. Selenium in Nutrition and Health. Nottingham University Press, Nottingham, UK, 2006.
33. Zilaee M, Ferns GA and Ghayour-Mobarhan M. Heat shock proteins and cardiovascular disease. Adv Clin Chem, 64, 2014, 73-115.
34. Garrido C, Gurbuxani S, Ravagnan L, Kroemer G. Heat shock proteins: endogenous modulators of apoptotic cell death. Biochem Biophys Res Commun, 286(3), 2001, 433-442.
35. Kennedy D, Jäger R, Mosser DD and Samali A. Regulation of apoptosis by heat shock proteins. IUBMB Life, 66(5), 2014, 327-338.
36. Mayer MP. Hsp70 chaperone dynamics and molecular mechanism. Trends Biochem Sci, 38(10), 2013, 507-514.
37. Shiber A and Ravid T. Chaperoning proteins for destruction: diverse roles of Hsp70 chaperones and their co-chaperones in targeting misfolded proteins to the proteasome. Biomolecules, 4(3), 2014, 704-724.
38. Duncan EJ, Cheetham ME, Chapple JP and van der Spuy J. The role of HSP70 and its co-chaperones in protein misfolding, aggregation and disease. Subcell Biochem, 78, 2015, 243-273.
39. Qu B, Jia Y, Liu Y, Wang H, Ren G and Wang H. The detection and role of heat shock protein 70 in various nondisease conditions and disease conditions: a literature review. Cell Stress & Chaperones, 2015. (In Press, Epub ahead of print]
40. Garrido C, Brunet M, Didelot C, Zermati Y, Schmitt E and Kroemer G. Heat shock proteins 27 and 70: anti-apoptotic proteins with tumorigenic properties. Cell Cycle, 5, 2006, 2592-2601.
41. Clerico EM, Tilitsky JM, Meng W and Gierasch LM. How hsp70 molecular machines interact with their substrates to mediate diverse physiological functions. J Mol Biol, 427, 2015, 1575-1588.
42. Ravagnan L, Gurbuxani S, Susin SA, Maisse C, Daugas E, Zamzami N, Mak T, Jäättelä M, Penninger JM, Garrido C and Kroemer GHeat-shock protein 70 antagonizes apoptosis-inducing factor. Nature Cell Biol, 3, 2001, 839-843.
43. Daugaard M, Rohde M and Jäättelä M. The heat shock protein 70 family: highly homologous proteins with overlapping and distinct functions. FEBS Lett, 581, 2007, 3702–3710.
44. Mahalka AK, Kirkegaard T, Jukola LT, Jäättelä M and Kinnunen PK. Human heat shock protein 70 (Hsp70) as a peripheral membrane protein. Biochim Biophys Acta, 1838, 2014, 1344-1361.
45. Meimaridou E, Gooljar SB and Chapple JP. From hatching to dispatching: the multiple cellular roles of the Hsp70 molecular chaperone machinery. J Mol Endocrinol, 42, 2009, 1-9.
46. Henstridge DC, Whitham M and Febbraio MA. Chaperoning to the metabolic party: The emerging therapeutic role of heat-shock proteins in obesity and type 2 diabetes. Mol Metab, 3, 2014, 781-793.
47. Pratt WB, Morishima Y, Peng HM and Osawa Y. Proposal for a role of the Hsp90/Hsp70-based chaperone machinery in making triage decisions when proteins undergo oxidative and toxic damage. Exp Biol Med (Maywood), 235, 2010, 278- 289.
48. Kelley PM and Schlesinger MJ. The effect of amino acid analogues and heat shock on gene expression in chicken embryo fibroblasts. Cell, 15(4), 1978, 1277-1286.
49. Kelley PM and Schlesinger MJ. Antibodies to two major chicken heat shock proteins cross-react with similar proteins in widely divergent species. Mol Cell Biol, 2(3), 1982, 267-274.
50. Voellmy R and Bromley PA. Massive heat-shock polypeptide synthesis in late chicken embryos: convenient system for study of protein synthesis in highly differentiated organisms. Mol Cell Biol, 2(5), 1982, 479-483.
51. Voellmy R, Bromley P, Kocher HP. Structural similarities between corresponding heat-shock proteins from different eukaryotic cells. J Biol Chem, 258(6), 1983, 3516-3522.
52. Morimoto R and Fodor E. Cell-specific expression of heat shock proteins in chicken reticulocytes and lymphocytes. J Cell Biol, 99(4 Pt 1), 1984, 1316-1323.
53. Banerji SS, Theodorakis NG and Morimoto RI. Heat shock-induced translational control of HSP70 and globin synthesis in chicken reticulocytes. Mol Cell Biol, 4(11), 1984, 2437-2448.
54. Morimoto RI, Hunt C, Huang SY, Berg KL and Banerji SS. Organization, nucleotide sequence, and transcription of the chicken HSP70 gene. J Biol Chem, 261(27), 1986, 12692-12699.
55. Banerji SS, Laing K and Morimoto RI. Erythroid lineage-specific expression and inducibility of the major heat shock protein HSP70 during avian embryogenesis. Genes, 1(9), 1987, 946-953.
56. Dash A, Chung S and Zelenka PS. Expression of HSP70 mRNA in the embryonic chicken lens: association with differentiation. Exp Eye Res, 58(4), 1994, 381-387.
57. Gabriel JE, Ferro JA, Stefani RM, Ferro MI, Gomes SL and Macari M. Effect of acute heat stress on heat shock protein 70 messenger RNA and on heat shock protein expression in the liver of broilers. Br Poult Sci, 37(2), 1996, 443-449.
58. Gabriel JE, da Mota AF, Boleli IC, Macari M and Coutinho LL. Effect of moderate and severe heat stress on avian embryonic hsp70 gene expression. Growth Dev Aging, 66(1), 2002, 27-33.
59. Leandro NS, Gonzales E, Ferro JA, Ferro MI, Givisiez PE and Macari M. Expression of heat shock protein in broiler embryo tissues after acute cold or heat stress. Mol Reprod Dev, 67(2), 2004, 172-177.
60. Surai PF, Noble RC, Speake BK. Tissue-specific differences in antioxidant distribution and susceptibility to lipid peroxidation during development of the chick embryo. Biochim Biophys Acta, 1304(1), 1996, 1-10.
61. Surai PF. Tissue-specific changes in the activities of antioxidant enzymes during the development of the chicken embryo. Br Poult Sci, 40(3), 1999, 397-405.
62. Zhen FS, Du HL, Xu HP, Luo QB and Zhang XQ. Tissue and allelic-specific expression of hsp70 gene in chickens: basal and heat-stress-induced mRNA level quantified with real-time reverse transcriptase polymerase chain reaction. Br Poult Sci, 47(4), 2006, 449-455.
63. Surai PF. Natural Antioxidants in Avian Nutrition and Reproduction. Nottingham University Press, Nottingham, UK, 2002.
64. Maak S, Melesse A, Schmidt R, Schneider F and Von Lengerken G. Effect of long-term heat exposure on peripheral concentrations of heat shock protein 70 (Hsp70) and hormones in laying hens with different genotypes. Br Poul Sci, 44(1), 2003, 133-138.
65. Figueiredo D, Gertler A, Cabello G, Decuypere E, Buyse J and Dridi S. Leptin downregulates heat shock protein-70 (HSP-70) gene expression in chicken liver and hypothalamus. Cell Tissue Res, 329(1), 2007, 91-101.
66. Gan JK, Zhang DX, He DL, Zhang XQ, Chen ZY and Luo QB. Promoter methylation negatively correlated with mRNA expression but not tissue differential expression after heat stress. Genet Mol Res, 12(1), 2013, 809-819.
67. Yu J, Bao E, Yan J and Lei L. Expression and localization of Hsps in the heart and blood vessel of heat-stressed broilers. Cell Stress Chaperones, 13(3), 2008, 327-335.
68. Zhang WW, Kong LN, Zhang XQ and Luo QB. Alteration of HSF3 and HSP70 mRNA expression in the tissues of two chicken breeds during acute heat stress. Genet Mol Res, 13(4), 2014, b9787-9794.
69. Gan JK, Jiang LY, Kong LN, Zhang XQ and Luo QB. Analysis of genetic diversity of the heat shock protein 70 gene on the basis of abundant sequence polymorphisms in chicken breeds. Genet Mol Res, 14(1), 2015, 1538-1545.
70. Hao Y, Gu XH and Wang XL. Overexpression of heat shock protein 70 and its relationship to intestine under acute heat stress in broilers: 1. Intestinal structure and digestive function. Poult Sci, 91(4), 2012, 781-789.
71. Gu XH, Hao Y and Wang XL. Overexpression of heat shock protein 70 and its relationship to intestine under acute heat stress in broilers: 2. Intestinal oxidative stress. Poult Sci, 91(4), 2012, 790-799.
72. Soleimani AF, Zulkifli I, Omar AR and Raha AR. Physiological responses of 3 chicken breeds to acute heat stress. Poult Sci, 90(7), 2011, 1435-1440.
73. Felver-Gant JN, Mack LA, Dennis RL, Eicher SD, Cheng HW. Genetic variations alter physiological responses following heat stress in 2 strains of laying hens. Poult Sci, 91(7), 2012, 1542-1551.
74. Gaviol HC, Gasparino E, Prioli AJ and Soares MA. Genetic evaluation of the HSP70 protein in the Japanese quail (Coturnix japonica). Genet Mol Res, 7(1), 2008, 133-139.
75. Xia M, Gan J, Luo Q, Zhang X snd Yang G. Identification of duck HSP70 gene, polymorphism analysis and tissue expression under control and heat stress conditions. Br Poult Sci, 54(5), 2013, 562-566.
76. Erlejman AG, Lagadari M, Toneatto J, Piwien-Pilipuk G and Galigniana MD. Regulatory role of the 90-kDa-heat-shock protein (Hsp90) and associated factors on gene expression. Biochim Biophys Acta, 1839(2), 2014, 71-87.
77. Karagöz GE and Rüdiger SG. Hsp90 interaction with clients. Trends Biochem Sci, 40(2), 2015, 117-125.
78. Mayer MP and Le Breton L. Hsp90: breaking the symmetry. Mol Cell, 58(1), 2015, 8-20.
79. Khurana N and Bhattacharyya S. Hsp90, the concertmaster: tuning transcription. Front Oncol, 5, 2015, 100.
80. Csermely P, Schnaider T, Soti C, Prohászka Z and Nardai G. The 90-kDa molecular chaperone family: structure, function, and clinical applications. A comprehensive review. Pharmacol Ther, 79(2), 1998, 129-168.
81. Bagatell R, Paine-Murrieta GD, Taylor CW, Pulcini EJ, Akinaga S, Benjamin IJ and Whitesell L. Induction of a heat shock factor 1-dependent stress response alters the cytotoxic activity of hsp90-binding agents. Clin Cancer Res, 6(8), 2000, 3312-3318.
82. Oksala NK, Ekmekçi FG, Ozsoy E, Kirankaya S, Kokkola T, Emecen G, Lappalainen J, Kaarniranta K and Atalay M. Natural thermal adaptation increases heat shock protein levels and decreases oxidative stress. Redox Biol, 3, 2014, 25-28.
83. Li J, Sun L, Xu C, Yu F, Zhou H, Zhao Y, Zhang J, Cai J, Mao C, Tang L, Xu Y and He J. Structure insights into mechanisms of ATP hydrolysis and the activation of human heat-shock protein 90. Acta Biochim Biophys Sinica, 44(4), 2012, 300-306.
84. Li J, Soroka J and Buchner J. The Hsp90 chaperone machinery: conformational dynamics and regulation by cochaperones. Biochim Biophys Acta, 1823(3), 2012, 624-635.
85. Sreedhar AS, Kalmár E, Csermely P and Shen YF. Hsp90 isoforms: functions, expression and clinical importance. FEBS Lett, 562(1-3), 2004, 11-15.
86. Revathi B and Prashanth K. Potential Hsp90 Inhibitors: A Novel Target for Cancer Therapy. Chemotherapy, 4, 2015, 146.
87. Jackson SE. Hsp90: structure and function. Top Curr Chem, 328, 2013, 155-240.
88. Garcia-Carbonero R, Carnero A and Paz-Ares L. Inhibition of HSP90 molecular chaperones: moving into the clinic. Lancet Oncol, 14(9), 2013, e358-369.
89. Wayne N, Mishra P and Bolon DN. Hsp90 and client protein maturation. Methods in Mol Biol, 787, 2011, 33-44.
90. Zhang H and Burrows F. Targeting multiple signal transduction pathways through inhibition of Hsp90. J Mol Med (Berlin, Germany), 82(8), 2004, 488-499.
91. Taipale M, Jarosz DF and Lindquist S. HSP90 at the hub of protein homeostasis: emerging mechanistic insights. Nature Rev Mol Cell Biol, 11(7), 2010, 515-528.
92. Trinklein ND, Chen WC, Kingston RE and Myers RM. Transcriptional regulation and binding of heat shock factor 1 and heat shock factor 2 to 32 human heat shock genes during thermal stress and differentiation. Cell Stress Chaperones, 9(1), 2004, 21-28.
93. Chiosis G, Vilenchik M, Kim J and Solit D. Hsp90: the vulnerable chaperone. Drug Discov Today, 9(20), 2004, 881- 888.
94. Hong DS, Banerji U, Tavana B, George GC, Aaron J and Kurzrock R. Targeting the molecular chaperone heat shock protein 90 (HSP90): lessons learned and future directions. Cancer Treat Rev, 39(4), 2013, 375-387.
95. Herring G and Gawlik DE. The role of stress proteins in the study of allostatic overload in birds: use and applicability to current studies in avian ecology. ScientificWorldJournal, 28(7), 2007. 1596-1602.
96. Barrott JJ and Haystead TA. Hsp90, an unlikely ally in the war on cancer. FEBS J, 280(6), 2013, 1381-1396.
97. Whitesell L and Lindquist SL. HSP90 and the chaperoning of cancer. Nature Rev Cancer, 5(10), 2005, 761-772.
98. Daniel S, Bradley G, Longshaw VM, Söti C, Csermely P and Blatch GL. Nuclear translocation of the phosphoprotein Hop (Hsp70/Hsp90 organizing protein) occurs under heat shock, and its proposed nuclear localization signal is involved in Hsp90 binding. Biochim Biophys Acta, 1783(6), 2008, 1003-1014.
99. Catelli MG, Binart N, Feramisco JR and Helfman DM. Cloning of the chick hsp 90 cDNA in expression vector. Nucleic Acids Res, 13(17), 1985, 6035-6047.
100.Catelli MG, Binart N, Jung-Testas I, Renoir JM, Baulieu EE, Feramisco J.R and Welch WJ. The common 90-kd protein component of non-transformed '8S' steroid receptors is a heat-shock protein. EMBO J, 4(12), 1985, 3131-3135.
101.Iannotti AM, Rabideau DA and Dougherty JJ. Characterization of purified avian 90,000-Da heat shock protein. Arch Biochem Biophys, 264(1), 1988, 54-60.
102.Binart N, Chambraud B, Dumas B, Rowlands DA, Bigogne C, Levin JM, Garnier J, Baulieu EE and Catelli MG. The cDNA-derived amino acid sequence of chick heat shock protein Mr 90,000 (HSP 90) reveals a "DNA like" structure: potential site of interaction with steroid receptors. Biochem Biophys Res Commun, 159(1), 1989, 140-147.
103.Radanyi C, Renoir JM, Sabbah M and Baulieu EE. Chick heat-shock protein of Mr =90,000, free or released from progesterone receptor, is in a dimeric form. J Biol Chem, 264(5), 1989, 2568-2573.
104.Vourch C, Binart N, Chambraud B, David JP, Jérôme V, Baulieu EE and Catelli M.G. Isolation and functional analysis of chicken 90-kDa heat shock protein gene promoter. Nucleic Acids Res, 17(13), 1989, 5259-5272.
105.Pekki AK. Different immunoelectron microscopic locations of progesterone receptor and HSP90 in chick oviduct epithelial cells. J Histochem Cytochem, 39(8), 1991, 1095-1101.
106.Czar MJ, Welsh MJ and Pratt WB. Immunofluorescence localization of the 90-kDa heat-shock protein to cytoskeleton. Eur J Cell Biol, 70(4), 1996, 322-330.
107.Passinen S, Valkila J, Manninen T, Syvälä H and Ylikomi T. The C-terminal half of Hsp90 is responsible for its cytoplasmic localization. Eur J Biochem, 268(20), 2001, 5337-5342.
108.Jérôme V, Vourch C, Baulieu EE and Catelli MG. Cell cycle regulation of the chicken hsp90 alpha expression. Exp Cell Res, 205(1), 1993, 44-51.
109.Jérôme V, Léger J, Devin J, Baulieu EE and Catelli MG. Growth factors acting via tyrosine kinase receptors induce HSP90 alpha gene expression. Growth Factors, 4(4), 1991, 317-327.
110.Meng X, Jérôme V, Devin J, Baulieu EE and Catelli MG. Cloning of chicken hsp90 beta: the only vertebrate hsp90 insensitive to heat shock. Biochem Biophys Res Commun, 190(2), 1993, 630-636.
111.Meng X, Baulieu EE and Catelli M.G. Isolation of chicken hsp90 beta gene promoter. Biochem Biophys Res Commun, 206(2), 1995, 644-651.
112.Grenert JP, Johnson BD and Toft DO. The importance of ATP binding and hydrolysis by hsp90 in formation and function of protein heterocomplexes. J Biol Chem, 274(25), 1999, 17525-17533.
113.Owen BA, Sullivan WP, Felts SJ and Toft DO. Regulation of heat shock protein 90 ATPase activity by sequences in the carboxyl terminus. J Biol Chem, 277(9), 2002, 7086-7091.
114.Bouhouche-Chatelier L, Chadli A and Catelli MG. The N-terminal adenosine triphosphate binding domain of Hsp90 is necessary and sufficient for interaction with estrogen receptor. Cell Stress Chaperones, 6(4), 2001, 297-305.
115.Garnier C, Lafitte D, Tsvetkov PO, Barbier P, Leclerc-Devin J, Millot JM, Briand C, Makarov AA, Catelli MG and Peyrot V. Binding of ATP to heat shock protein 90: evidence for an ATP-binding site in the C-terminal domain. J Biol Chem, 277(14), 2002, 12208-12214.
116.Johnson BD, Chadli A, Felts SJ, Bouhouche I, Catelli MG and Toft DO. Hsp90 chaperone activity requires the fulllength protein and interaction among its multiple domains. J Biol Chem, 275(42), 2000, 32499-32507.
117.Lei L, Yu J and Bao E. Expression of heat shock protein 90 (Hsp90) and transcription of its corresponding mRNA in broilers exposed to high temperature. Br Poult Sci, 50(4), 2009, 504-511.
118.Sass JB and Krone PH. HSP90alpha gene expression may be a conserved feature of vertebrate somitogenesis. Exp Cell Res, 233(2), 1997, 391-394.
119.Holley SJ and Yamamoto KR. A role for Hsp90 in retinoid receptor signal transduction. Mol Biol Cell, 6(12), 1995, 1833-1842.
120.Kim HR, Kang KI, Kang HS and Kim HD. Identification of heat shock protein 90-associated 84-kDa phosphoprotein. J Biochem, 126(6), 1999, 1025-1032.
121.Marcu MG, Chadli A, Bouhouche I, Catelli M and Neckers LM. The heat shock protein 90 antagonist novobiocin interacts with a previously unrecognized ATP-binding domain in the carboxyl terminus of the chaperone. J Biol Chem, 275(47), 2000, 37181-37186.
122.Shinozaki F, Minami M, Chiba T, Suzuki M, Yoshimatsu K, Ichikawa Y, Terasawa K, Emori Y, Matsumoto K, Kurosaki T, Nakai A, Tanaka K and Minami Y. Depletion of hsp90beta induces multiple defects in B cell receptor signaling. J Biol Chem, 281(24), 2006, 16361-16369.
123.Bhat A, Gomis S, Potter A and Tikoo SK. Role of Hsp90 in CpG ODN mediated immunostimulation in avian macrophages. Mol Immunol, 47(6), 2010 1337-1346.
124.Nagahori K, Iwamoto S, Maruyama A, Suzuki S, Hosomichi K, Shiina T, Hara H, Yoshida Y and Hanzawa K. Basic characterization of 90 kDa heat shock protein genes HSP90AA1, HSP90AB1, HSP90B1 and TRAP1 expressed in Japanese quail (Coturnix japonica). Anim Sci J, 81(4), 2010, 513-518.
125.Wu ML, Ho YC, Lin CY and Yet SF. Heme oxygenase-1 in inflammation and cardiovascular disease. Am J Cardiovasc Dis, 1(2), 2011, 150-158.
126.Fredenburgh LE, Merz AA and Cheng S. Haeme oxygenase signalling pathway: implications for cardiovascular disease. Eur Heart J, 36(24), 2015, 1512-1518.
127.Soares MP and Bach FH. Heme oxygenase-1: from biology to therapeutic potential. Trends Mol Med, 15(2), 2009, 50- 58.
128.Ryter SW and Choi AM. Targeting heme oxygenase-1 and carbon monoxide for therapeutic modulation of inflammation. Translation Res, 2015 (In Press, Epub ahead of print).
129.Durante W. Targeting heme oxygenase-1 in vascular disease. Curr Drug Targets, 11(12), 2010, 1504-1516.
130.Haines DD, Lekli I, Teissier P, Bak I and Tosaki A. Role of haeme oxygenase-1 in resolution of oxidative stress-related pathologies: focus on cardiovascular, lung, neurological and kidney disorders. Acta Physiol (Oxford, England), 204(4), 2012, 487-501.
131.Zahir F, Rabbani G, Khan RH, Rizvi SJ, Jamal MS and Abuzenadah AM. The pharmacological features of bilirubin: the question of the century. Cell Mol Biol Lett, 20(3), 2015, 418-447.
132.Bilban M, Haschemi A, Wegiel B, Chin BY, Wagner O and Otterbein LE. Heme oxygenase and carbon monoxide initiate homeostatic signaling. J Mol Med (Berlin, Germany), 86(3), 2008, 267-279.
133.Pae HO and Chung HT. Heme oxygenase-1: its therapeutic roles in inflammatory diseases. Immune Network, 9(1), 2009, 12-19.
134.Morse D, Lin L, Choi AM and Ryter SW. Heme oxygenase-1, a critical arbitrator of cell death pathways in lung injury and disease. Free Radic Biol Med, 47(1), 2009, 1-12.
135.Kapturczak MH, Wasserfall C, Brusko T, Campbell-Thompson M, Ellis TM, Atkinson MA and Agarwal A. Heme oxygenase-1 modulates early inflammatory responses: evidence from the heme oxygenase-1-deficient mouse. Am J Pathol, 165(3), 2004, 1045–1053.
136.Poss KD and Tonegawa S. 1997. Reduced stress defense in heme oxygenase 1-deficient cells. Proc Natl Acad Sci USA, 94(20), 1997, 10925–10930.
137.Tzima S, Victoratos P, Kranidioti K, Alexiou M, Kollias G. Myeloid heme oxygenase–1 regulates innate immunity and autoimmunity by modulating IFN-b production. J Exp Med, 206(5), 2009, 1167–1179.
138.Vachharajani TJ, Work J, Issekutz AC and Granger DN. Heme oxygenase modulates selectin expression in different regional vascular beds. Am J Physiol Heart Circ Physiol, 278(5), 2000, H1613–H1617.
139.True AL, Olive M, Boehm M, San H, Westrick RJ, Raghavachari N, Xu X, Lynn EG, Sack MN and Munson PJ. Heme oxygenase-1 deficiency accelerates formation of arterial thrombosis through oxidative damage to the endothelium, which is rescued by inhaled carbon monoxide. Circulation Res, 101(9), 2007, 893–901.
140.Wegiel B, Nemeth Z, Correa-Costa M, Bulmer AC and Otterbein LE. Heme oxygenase-1: a metabolic nike. Antioxid Redox Signal, 20(11), 2014, 1709-1722.
141.Yet SF, Perrella MA, Layne MD, Hsieh CM, Maemura K, Kobzik L, Wiesel P, Christou H, Kourembanas S and Lee ME. Hypoxia induces severe right ventricular dilatation and infarction in heme oxygenase-1 null mice. J Clin Invest, 103, 1999, R23–29.
142.Liu X, Wei J, Peng DH, Layne MD and Yet SF. Absence of heme oxygenase-1 exacerbates myocardial ischemia/reperfusion injury in diabetic mice. Diabetes, 54, 2005, 778–784.
143.Dennery PA. Regulation and role of heme oxygenase in oxidative injury. Curr Top Cell Regul, 36, 2000, 181–199.
144.Schipper HM and Song W. A heme oxygenase-1 transducer model of degenerative and developmental brain disorders. Int J Mol Sci, 16(3), 2015, 5400-5419.
145.Chang AY, Chan JY, Cheng H-L, Tsai C-Y and Chan SH. Hypoxia-inducible factor 1/heme oxygenase 1 cascade as upstream signals in the prolife role of heat shock protein 70 at rostral ventrolateral medulla during experimental brain stem death. Shock, 32(6), 2009, 651–658.
146.Wegiel B, Hedblom A, Li M, Gallo D, Csizmadia E, Harris C, Nemeth Z, Zuckerbraun B, Soares M and Persson JL. Heme oxygenase-1 derived carbon monoxide permits maturation of myeloid cells. Cell Death Dis, 5(3), 2014, e1139.
147.Terry CM, Clikeman JA, Hoidal JR and Callahan KS. Effect of tumor necrosis factor-a and interleukin-1a on heme oxygenase-1 expression in human endothelial cells. Am J Physiol Heart Circ Physiol, 274(3), 1998, H883–H891.
148.Niess AM, Passek F, Lorenz I, Schneider EM, Dickhuth H-H, Northoff H and Fehrenbach E. Expression of the antioxidant stress protein heme oxygenase-1 (HO-1) in human leukocytes: acute and adaptational responses to endurance exercise. Free Radic Biol Med, 26(1), 1999, 184–192.
149.Immenschuh S, Tan M and Ramadori G. Nitric oxide mediates the lipopolysaccharide dependent upregulation of the heme oxygenase-1 gene expression in cultured rat Kupffer cells. J Hepatol, 30(1), 1999, 61–69.
150.Bissell DM, Hammaker L and Schmid R. Liver sinusoidal cells Identification of a subpopulation for erythrocyte catabolism. J Cell Biol, 54(1), 1972, 107–119.
151.Chauveau C, Remy S, Royer PJ, Hill M, Tanguy-Royer S, Hubert F-X, Tesson L, Brion R, Beriou G and Gregoire M. Heme oxygenase-1 expression inhibits dendritic cell maturation and proinflammatory function but conserves IL-10 expression. Blood, 106(5), 2005, 1694–1702.
152.Lee T-S and Chau L-Y. Heme oxygenase-1 mediates the anti-inflammatory effect of interleukin-10 in mice. Nature Med, 8(3), 2002, 240–246
153.Wijayanti N, Huber S, Samoylenko A, Kietzmann T and Immenschuh S. Role of NF-kB and p38 MAP kinase signaling pathways in the lipopolysaccharide-dependent activation of heme oxygenase-1 gene expression. Antioxid Redox Signal, 6(5), 2004, 802–810.
154.Remy S, Blancou P, Tesson L, Tardif V, Brion R, Royer PJ, Motterlini R, Foresti R, Painchaut M and Pogu S. Carbon monoxide inhibits TLR-induced dendritic cell immunogenicity. J Immunol, 182(4), 2009, 1877–1884.
155.George JF, Braun A, Brusko TM, Joseph R, Bolisetty S, Wasserfall CH, Atkinson MA, Agarwal A and Kapturczak MH. Suppression by CD4+CD25+ regulatory t cells is dependent on expression of heme oxygenase-1 in antigen-presenting cells. Am J Pathol, 173(1), 12008, 54–160.
156.Moreau A, Hill M, Thebault P, Deschamps J, Chiffoleau E, Chauveau C, Moullier P, Anegon I, Alliot-Licht B and Cuturi M. Tolerogenic dendritic cells actively inhibit T cells through heme oxygenase-1 in rodents and in nonhuman primates. FASEB J, 23(9), 2009, 3070–3077.
157.Nakamichi I, Habtezion A, Zhong B, Contag CH, Butcher E.C and Omary MB. Hemin-activated macrophages home to the pancreas and protect from acute pancreatitis via heme oxygenase-1 induction. J Clin Invest, 115(11), 2005, 3007– 3014.
158.Choi KM, Kashyap PC, Dutta N., Stoltz GJ, Ordog T, Shea Donohue T, Bauer AJ, Linden DR, Szurszewski JH and Gibbons SJ. CD206-positive M2 macrophages that express heme oxygenase-1 protect against diabetic gastroparesis in mice. Gastroenterology, 138(7), 2010, 2399–2409.
159.Igarashi K and Sun J. The heme-Bach1 pathway in the regulation of oxidative stress response and erythroid differentiation. Antioxid Redox Signal, 8(1–2), 2006, 107–118.
160.Jang JS, Piao S, Cha Y-N and Kim C. Taurine chloramine activates Nrf2, increases HO-1 expression and protects cells from death caused by hydrogen peroxide. J Clin Biochem Nutr, 45(1), 2009, 37-43.
161.Semenza GL. Hypoxia-inducible factor 1: regulator of mitochondrial metabolism and mediator of ischemic preconditioning. Biochim Biophys Acta, 1813, 2010, 1263–1268.
162.Ndisang JF. Cross-talk between heme oxygenase and peroxisome proliferator-activated receptors in the regulation of physiological functions. Front Biosci (Landmark Ed), 19, 2014, 916-935.
163.De Backer O, Elinck E, Blanckaert B, Leybaert L, Motterlini R and Lefebvre RA. Water-soluble CO-releasing molecules Hemeoxygenase-1 in gastrointestinal diseases reduce the development of postoperative ileus via modulation of MAPK/HO-1 signalling and reduction of oxidative stress. Gut, 58(3), 2009, 347–356.
164.Soares MP, Usheva A, Brouard S, Berberat PO, Gunther L, Tobiasch E and Bach FH. Modulation of endothelial cell apoptosis by heme oxygenase-1-derived carbon monoxide. Antioxid Redox Signal, 4(2), 2002, 321-329.
165.Salinas M, Wang J, Rosa de Sagarra M, Martin D, Rojo AI, Martin-Perez J, Ortiz de Montellano PR and Cuadrado A. Protein kinase Akt/PKB phosphorylates heme oxygenase-1 in vitro and in vivo. FEBS Lett, 578(1), 2004, 90–94.
166.Bonkovsky HL, Healey JF and Pohl J. Purification and characterization of heme oxygenase from chick liver. Comparison of the avian and mammalian enzymes. Eur J Biochem, 189(1), 1990, 155-166.
167.Evans CO, Healey JF, Greene Y and Bonkovsky HL. Cloning, sequencing and expression of cDNA for chick liver haem oxygenase. Comparison of avian and mammalian cDNAs and deduced proteins. Biochem J, 273(Pt 3), 1991, 659-666.
168.Lu TH, Lambrecht RW, Pepe J, Shan Y, Kim T and Bonkovsky HL. Molecular cloning, characterization, and expression of the chicken heme oxygenase-1 gene in transfected primary cultures of chick embryo liver cells. Gene, 207(2), 1998, 177-186.
169.Shan Y, Pepe J, Lambrecht RW and Bonkovsky HL. Mapping of the chick heme oxygenase-1 proximal promoter for responsiveness to metalloporphyrins. Arch Biochem Biophys, 399(2), 2002, 159-166.
170.Shan Y, Lambrecht RW and Bonkovsky HL. Identification of key elements that are responsible for heme-mediated induction of the avian heme oxygenase-1 gene. Biochim Biophys Acta, 1679(2), 2004, 87-94.
171.Greene YJ, Healey JF and Bonkovsky HL. Immunochemical studies of haem oxygenase. Preparation and characterization of antibodies to chick liver haem oxygenase and their use in detecting and quantifying amounts of haem oxygenase protein. Biochem J, 279(3), 1991, 849-854.
172.Cable EE, Cable JW and Bonkovsky HL. Repression of hepatic delta-aminolevulinate synthase by heme and metalloporphyrins: relationship to inhibition of heme oxygenase. Hepatology, 18(1), 1993, 119-127.
173.Gabis KK, Gildemeister OS, Pepe JA, Lambrecht RW and Bonkovsky HL. Induction of heme oxygenase-1 in LMH cells. Comparison of LMH cells to primary cultures of chick embryo liver cells. Biochim Biophys Acta, 1290(1), 1996, 113-120.
174.Sardana MK, Sassa S and Kappas A. Hormonal regulation of heme oxygenase induction in avian hepatocyte culture. Biochem Pharmacol, 34(16), 1985, 2937-2944.
175.Maines MD and Sinclair P. Cobalt regulation of heme synthesis and degradation in avian embryo liver cell culture. J Biol Chem, 252(1), 1977, 219-223.
176.Sardana MK, Drummond GS, Sassa S and Kappas A. The potent heme oxygenase inducing action of arsenic and parasiticidal arsenicals. Pharmacology, 23(5), 1981, 247-253.
177.Sardana MK, Sassa S and Kappas A. Metal ion-mediated regulation of heme oxygenase induction in cultured avian liver cells. J Biol Chem, 257(9), 1982, 4806-4811.
178.Prasad AR and Datta K. Altered regulation of hepatic heme metabolism in cadmium exposed chick embryo. Biochem Int, 8(2), 1984, 289-298.
179.Lincoln BC, Healey JF and Bonkovsky HL. Regulation of hepatic haem metabolism. Disparate mechanisms of induction of haem oxygenase by drugs and metals. The Biochem J, 250(1), 1988, 189-196.
180.Cable E, Greene Y, Healey J, Evans CO and Bonkovsky H. Mechanism of synergistic induction of hepatic heme oxygenase by glutethimide and iron: studies in cultured chick embryo liver cells. Biochem Biophys Res Commun, 168(1), 1990, 176-181.
181.Lu TH, Pepe JA, Gildemeister OS, Tyrrell RM and Bonkovsky HL. Regulation of expression of the human heme oxygenase-1 gene in transfected chick embryo liver cell cultures. Biochim Biophys Acta, 1352(3), 1997, 293-302.
182.Jacobs JM, Marek D, Walton HS, Sinclair PR and Sinclair J.F. Effect of sodium arsenite on heme metabolism in cultured chick embryo hepatocytes. Arch Biochem Biophys, 371(1), 1999, 8-14.
183.Elbirt KK, Whitmarsh AJ, Davis R and Bonkovsky HL. Mechanism of sodium arsenite-mediated induction of heme oxygenase-1 in hepatoma cells. Role of mitogen-activated protein kinases. J Biol Chem, 273(15), 1998, 8922-8931.
184.Shan Y, Lambrecht RW, Hong Lu T and Bonkovsky HL. Effects of phenylarsine oxide on expression of heme oxygenase-1 reporter constructs in transiently transfected cultures of chick embryo liver cells. Arch Biochem Biophys, 372(2), 1999, 224-229.
185.Gildemeister OS, Pepe JA, Lambrecht RW and Bonkovsky HL. Induction of heme oxygenase-1 by phenylarsine oxide. Studies in cultured primary liver cells. Mol Cell Biochem, 226(1-2), 2001, 17-26.
186.Lu TH, Shan Y, Pepe J, Lambrecht RW and Bonkovsky HL. Upstream regulatory elements in chick heme oxygenase-1 promoter: a study in primary cultures of chick embryo liver cells. Mol Cell Biochem, 209(1-2), 2000, 17-27.
187.Fernandez M and Bonkovsky HL. Vascular endothelial growth factor increases heme oxygenase-1 protein expression in the chick embryo chorioallantoic membrane. Br J Pharmacol, 139(3), 2003, 634-640.
188.Lincoln BC, Aw TY and Bonkovsky HL. Heme catabolism in cultured hepatocytes: evidence that heme oxygenase is the predominant pathway and that a proportion of synthesized heme is converted rapidly to biliverdin. Biochim Biophys Acta, 992(1), 1989, 49-58.
189.Druyan S, Cahaner A and Ashwell CM. The expression patterns of hypoxia-inducing factor subunit alpha-1, heme oxygenase, hypoxia upregulated protein 1, and cardiac troponin T during development of the chicken heart. Poult Sci, 86(11), 2007, 2384-2389.
190.Barbagallo I, Galvano F, Frigiola A, Cappello F, Riccioni G, Murabito P, D'Orazio N, Torella M, Gazzolo D and Li Volti G. Potential therapeutic effects of natural heme oxygenase-1 inducers in cardiovascular diseases. Antioxid Redox Signal, 18(5), 2013, 507-521.
191.Murakami A. Dose-dependent functionality and toxicity of green tea polyphenols in experimental rodents. Arch Biochem Biophys, 557, 2014, 3-10.
192.Mueller K, Blum NM, Kluge H and Mueller AS. Influence of broccoli extract and various essential oils on performance and expression of xenobiotic- and antioxidant enzymes in broiler chickens. Br J Nutr, 108(4), 2012, 588-602.
193.Peng YZ, Wang YW, Ning D and Guo YM. Changes of haematic parameters, redox status and mitochondrial complex activity in the heart and liver of broilers fed with different density diets under low ambient temperature. Avian Pathol, 42(4), 2013, 327-334.
194.Bag J. Regulation of heat-shock protein synthesis in chicken muscle culture during recovery from heat shock. Eur J Biochem, 135(3), 1983, 373-378.
195.Bag J. Free messenger ribonucleoprotein complexes of chicken primary muscle cells following modification of protein synthesis by heat-shock treatment. Eur J Biochem, 135(2), 1983, 187-196.
196.White CN and Hightower LE. Stress mRNA metabolism in canavanine-treated chicken embryo cells. Mol Cell Biol, 4(8), 1984, 1534-1541.
197.Collier NC and Schlesinger MJ. Induction of heat-shock proteins in the embryonic chicken lens. Exp Eye Res, 43(1), 1986, 103-117.
198.Collier NC and Schlesinger MJ. The dynamic state of heat shock proteins in chicken embryo fibroblasts. J Cell Biol, 103(4), 1986, 1495-1507.
199.Atkinson BG, Dean RL and Blaker TW. Heat shock induced changes in the geneexpression of terminally differentiating avian red blood cells. Can J Genet Cytol, 28(6), 1986, 1053-1063.
200.Guerriero V Jr and Raynes DA. Synthesis of heat stress proteins in lymphocytes from livestock. J Anim Sci, 68(9), 1990, 2779-2883.
201.Miller L and Qureshi MA. Heat-shock protein synthesis in chicken macrophages: influence of and in vitro heat shock, lead acetate, and lipopolysaccharide. Poult Sci, 71(6), 1992, 988-998.
202.Miller L and Qureshi MA. Comparison of heat-shock-induced and lipopolysaccharide-induced protein changes and tumoricidal activity in a chicken mononuclear cell line. Poult Sci, 71(6), 1992, 979-987.
203.Miller L and Qureshi MA. Molecular changes associated with heat-shock treatment in avian mononuclear and lymphoid lineage cells. Poult Sci, 71(3), 1992, 473-481.
204.Miller L and Qureshi MA. Induction of heat-shock proteins and phagocytic function of chicken macrophage following in vitro heat exposure. Vet Immunol Immunopathol, 30(2-3), 1992, 179-191.
205.Edens FW, Hill CH and Wang S. Heat shock protein response in phosphorus-deficient heat-stressed broiler chickens. Comp Biochem Physiol B, 103(4), 1992, 827-831.
206.Mezquita B, Mezquita C and Mezquita J. Marked differences between avian and mammalian testicular cells in the heat shock induction and polyadenylation of Hsp70 and ubiquitin transcripts. FEBS Lett, 436(3), 1998, 382-386.
207.Zulkifli I, Che Norma MT, Israf DA and Omar AR. The effect of early-age food restriction on heat shock protein 70 response in heat-stressed female broiler chickens. Br Poult Sci, 43(1), 2002, 141-145.
208.Wolfe MS and Zatz M. Synthesis of heat shock proteins in cultured chick pineal cells. Brain Res, 662(1-2), 1994, 273- 277.
209.Mahmoud K Z, Edens FW, Eisen EJ and Havenstein GB. The effect of dietary phosphorus on heat shock protein mRNAs during acute heat stress in male broiler chickens (Gallus gallus). Comp Biochem Physiol C Toxicol Pharmacol, 137, 2004, 11–18.
210.Xie J, Tang L, Lu L, Zhang L, Xi L, Liu HC, Odle J and Luo X. Differential expression of heat shock transcription factors and heat shock proteins after acute and chronic heat stress in laying chickens (Gallus gallus). PLoS One, 9(7), 2014, e102204.
211.Wang SH, Cheng CY, Chen CJ, Chen HH, Tang PC, Chen CF, Lee YP and Huang SY. Changes in protein expression in testes of L2 strain Taiwan country chickens in response to acute heat stress. Theriogenology, 82(1), 2014, 80-94.
212.Zuo J, Xu M, Abdullahi YA, Ma L, Zhang Z and Feng D. Constant heat stress reduces skeletal muscle protein deposition in broilers. J Sci Food Agric, 95(2), 2015, 429-436.
213.Hao Y and Gu XH. Effects of heat shock protein 90 expression on pectoralis major oxidation in broilers exposed to acute heat stress. Poult Sci, 93(11), 2014, 2709-2717.
214.Sun H, Jiang R, Xu S, Zhang Z, Xu G, Zheng J and Qu L. Transcriptome responses to heat stress in hypothalamus of a meat-type chicken. J Anim Sci Biotechnol, 6(1), 2015, 6.
215.Wang SH, Cheng CY, Tang PC, Chen CF, Chen HH, Lee YP and Huang SY. Differential gene expressions in testes of L2 strain Taiwan country chicken in response to acute heat stress. Theriogenology, 79(2), 2013, 374-382.
216.Luo QB, Song XY, Ji CL, Zhang XQ and Zhang DX. Exploring the molecular mechanism of acute heat stress exposure in broiler chickens using gene expression profiling. Gene, 546(2), 2014, 200-205.
217.Calabrese EJ, Dhawan G, Kapoor R, Iavicoli I, Calabrese V. What is hormesis and its relevance to healthy aging and longevity? Biogerontology, 2015 (In Press, Epub ahead of print).
218.Calabrese V, Dattilo S, Petralia A, Parenti R, Pennisi M, Koverech G, Calabrese V, Graziano A, Monte I, Maiolino L, Ferreri T, Calabrese EJ. Analytical approaches to the diagnosis and treatment of aging and aging-related disease: redox status and proteomics. Free Radic Res, 49(5), 2015, 511-524.
219.Spiro IJ, Sapareto SA, Raaphorst GP and Dewey WC. The effect of chronic and acute heat conditioning on the development of thermal tolerance. Int J Radiat Oncol Biol Phys, 8(1), 1982, 53-58.
220.Webb DR. Thermal tolerance of avian embryos: a review. Condor, 89, 1987, 874–898.
221.Decuypere E. Incubation temperature in relation to postnatal performance in chickens. Arch Exp Veterinarmed, 38(3), 1984, 439-449.
222.Minne B and Decuypere E. Effects of late prenatal temperatures on some thermoregulatory aspects in young chickens. Arch Exp Veterinarmed, 38(3), 1984, 374-383.
223.Piestun Y, Shinder D, Ruzal M, Halevy O, Brake J and Yahav S. Thermal manipulations during broiler embryogenesis: effect on the acquisition of thermotolerance. Poult Sci, 87(8), 2008, 1516-1525.
224.Piestun Y, Harel M, Barak M, Yahav S and Halevy O. Thermal manipulations in late-term chick embryos have immediate and longer term effects on myoblast proliferation and skeletal muscle hypertrophy. J Appl Physiol, 106, 2009, 233–240.
225.Loyau T, Berri C, Bedrani L, Métayer-Coustard S, Praud C , Duclos MJ, Tesseraud S, Rideau N, Everaert N, Yahav S, Mignon-Grasteau S and Collin A. Thermal manipulation of the embryo modifies the physiology and body composition of broiler chickens reared in floor pens without affecting breast meat processing quality. J Anim Sci, 91(8), 2013, 3674- 3685.
226.Piestun Y, Druyan S, Brake J and Yahav S. Thermal manipulations during broiler incubation alter performance of broilers to 70 days of age. Poult Sci, 92(5), 2013, 1155-1163.
227.Janisch S, Sharifi AR, Wicke M and Krischek C. Changing the incubation temperature during embryonic myogenesis influences the weight performance and meat quality of male and female broilers. Poult Sci, 2015. (In Press, Epub ahead of print).
228.Piestun Y, Zimmerman I and Yahav S. Thermal manipulations of turkey embryos: The effect on thermoregulation and development during embryogenesis. Poult Sci, 94(2), 2015, 273-280.
229.Piestun Y, Yahav S and Halevy O. Thermal manipulation during embryogenesis affects myoblast proliferation and skeletal muscle growth in meat-type chickens. Poult Sci, 2015 (In Press, Epub ahead of print).
230.Moraes VMB, Malheirosb RD, Bruggeman V, Collin A, Tona K, Van As P, Onagbesan OM, Buyse J, Decuypere E and Macari M. The effect of timing of thermal conditioning during incubation on embryo physiological parameters and its relationship to thermotolerance in adult broiler chickens. J Therm Biol, 29(1), 2004, 55–61.
231.Yahav S, Sasson Rath R and Shinder D.. The effect of thermal manipulations during embryogenesis of broiler chicks (Gallus domesticus) on hatchability, body weight and thermoregulation after hatch. J Therm Biol, 29, 2004, 245–250.
232.Yahav S, Collin A, Shinder D and Picard M. 2004. Thermal manipulations during broiler embryogenesis: effects of timing and temperature. Poult Sci, 83, 245–250.
233.Collin A, Picard M and Yahav S. The effect of duration of thermal manipulation during chick's embryogenesis on body weight and body temperature of post hatched chicks. Anim Res, 54, 2005, 105–112.
234.Collin A, Berri C, Tesseraud S, Rodon FER, Skiba-Cassy S, Crochet S, Duclos MJ, Rideau N, Tona K, Buyse J, Bruggeman V, Decuypere E, Picard M and Yahav S.. Effects of thermal manipulation during early and late embryogenesis on thermotolerance and breast muscle characteristics in broiler chickens. Poult Sci, 86, 2007, 795–800.
235.Tona K, Onagbesan O, Bruggeman V, Collin A, Berri C, Duclos M,Tesseraud S, Buyse J, Decuypere E and Yahav S. Effects of heat conditioning at d16–18 of incubation or during early broiler rearing on embryophysiology, post-hatch growth performance and heat tolerance. Arch Geflug, 72, 2008, 75–83.
236.Yalçin S, Çabuk M, Bruggeman V, Babacanoglu E, Buyse J, Decuypere E and Siegel PB. Acclimation to heat during incubation. 1. Embryonic morphological traits, blood biochemistry, and hatching performance. Poult Sci, 87, 2008, 1219–1228.
237.Yalçin S,Özkan S, Sigel PB, Yenisey Ç and Aksit M. Manipulation of in cubation temperatures to increase cold resistance of broilers: Influence on embryo development, organ weights, hormones and body composition. Japan Poult Sci, 49, 2012, 133–139.
238.Walstra I, ten Napel J, Kemp B and van den Brand H. Temperature manipulation during layer chick embryogenesis. Poult Sci, 89, 2010, 1502–1508.
239.Lourens A, van den Brand H, Meijerhof R and Kemp B. Effect of eggshell temperature during incubation on embryo development, hatchability, and posthatch development. Poult Sci, 84, 2005, 914–920.
240.Werner C, Wecke C, Liebert F and Wicke M. Increasing the incubation temperature between embryonic day 7 and 10 has no influence on the growth and slaughter characteristics as well as meat quality of broilers. Animal, 4, 2010, 810– 816.
241.Krischek C, Kuembet U, Wicke M and Gerken M. A higher incubation temperature between embryonic day 3 and 6 influences growth and meat quality characteristics of broiler after hatch. Eur Poult Sci, 77, 2013, 59–65.
242.Kamanli S, Durmus I, Yalçin S, Yildirim U and Meral Ö. Effect of prenatal temperature conditioning of laying hen embryos: Hatching, live performance and response to heat and cold stress during laying period. J Therm Biol, 51, 2015, 96-104.
243.Sozcu A and Ipek A. Acute and chronic eggshell temperature manipulations during hatching term influence hatchability, broiler performance, and ascites incidence. Poult Sci, 94(2), 2015, 319-327.
244.Loyau T, Bedrani L, Berri C, Métayer-Coustard S, Praud C, Coustham V, Mignon-Grasteau S, Duclos MJ, Tesseraud S, Rideau N, Hennequet-Antier N, Everaert N, Yahav S and Collin A. Cyclic variations in incubation conditions induce adaptive responses to later heat exposure in chickens: a review. Animal, 9, 2015, 76-85.
245.Li C, Guo S, Zhang M, Gao J and Guo Y. DNA methylation and histone modification patterns during the late embryonic and early postnatal development of chickens. Poult Sci, 94(4), 2015, 706-721.
246.Liu H, Liu J, Yan X, Li Q, Zhao Y, Wang Y, Zhang R, Wang G, Wang H, Li X, Yang C, Li L, Han C and Wang J. Impact of thermal stress during incubation on gene expression in embryonic muscle of Peking Ducks (Anasplatyrhynchos domestica). J Therm Biol, 2015 (In Press)
247.Sun L, Lamont SJ, Cooksey AM, McCarthy F, Tudor CO, Vijay-Shanker K, De Rita RM, Rothschild M, Ashwell C, Persia ME and Schmidt CJ.. Transcriptome response to heat stress in a chicken hepatocellular carcinoma cell line. Cell Stress Chaperones, 2015 (In Press, Epub ahead of print).
248.Yahav S, Shamai A, Haberfeld A, Horev G, Hurwitz S and Einat M. Induction of thermotolerance in chickens by temperature conditioning: heat shock protein expression. Ann N Y Acad Sci, 813, 1997, 628-636.
249.Yahav S, Shamay A, Horev G, Bar-Ilan D, Genina O and Friedman-Einat M. Effect of acquisition of improved thermotolerance on the induction of heat shock proteins in broiler chickens. Poult Sci, 76(10), 1997, 1428-1434.
250.Wang S and Edens FW. Heat conditioning induces heat shock proteins in broiler chickens and turkey poults. Poult Sci, 77(11), 1998, 1636-1645.
251.Givisiez PE, Ferro JA, Ferro MI, Kronka SN, Decuypere E and Macari M. Hepatic concentration of heat shock protein 70 kD (Hsp70) in broilers subjected to different thermal treatments. Br PoultSci, 40(2), 1999, 292-296.
252.Liew PK, Zulkifli I, Hair-Bejo M, Omar A.R and Israf DA. Effects of early age feed restriction and heat conditioning on heat shock protein 70 expression, resistance to infectious bursal disease, and growth in male broiler chickens subjected to heat stress. Poult Sci, 82(12), 2003, 1879-1885.
253.Al-Zghoul MB, Ismail ZB, Dalab AE, Al-Ramadan A, Althnaian TA, Al-Ramadan SY, Ali AM, Albokhadaim IF, Al Busadah KA, Eljarah A, Jawasreh KI and Hannon KM. Hsp90, Hsp60 and HSF-1 genes expression in muscle, heart and brain of thermally manipulated broiler chicken. Res Vet Sci, 99, 2015, 105-111.
254.Rajkumar U, Vinoth A, Shanmugam M, Rajaravindra KS and Rama Rao SV. Effect of Embryonic Thermal Exposure on Heat Shock Proteins (HSPs) Gene Expression and Serum T3 Concentration in Two Broiler Populations. Anim Biotechnol, 26(4), 2015, 260-267.
255.Widelitz RB, Magun BE and Gerner E.W. Effects of cycloheximide on thermotolerance expression, heat shock protein synthesis, and heat shock protein mRNA accumulation in rat fibroblasts. Mol Cell Biol, 6, 1986, 1088–1094.
256.Aujame L and Firko H. The major inducible heat shock protein hsp68 is not required for acquisition of thermal resistance in mouse plasmacytoma cell lines. Mol Cell Biol, 8, 1988, 5486–5494.
257.Oommen D, Giricz Z, Srinivas UK and Samali A. Atypical heat shock response and acquisition of thermotolerance in P388D1 cells. Biochem Biophys Res Communicat, 430(1), 2013, 236-240.
258.Horowitz M. Heat acclimation, epigenetics, and cytoprotection memory. Compr Physiol, 4(1), 2014, 199-230.
259.Das S, Pan D, Bera AK, Rana T, Bandyopadhyay S, De S, Das SK, Bhattacharya D and Bandyopadhyay SK. Stress inducible heat shock protein 70: a potent molecular and toxicological signature in arsenic exposed broiler chickens. Mol Biol Rep, 37(7), 2010, 3151-3155.
260.Guo Y, Zhao P, Guo G, Hu Z, Tian L, Zhang K, Sun Y, Zhang X, Zhang W and Xing M. Effects of Arsenic Trioxide Exposure on Heat Shock Protein Response in the Immune Organs of Chickens. Biol Trace Elem Res, 2015. (In Press; Epub ahead of print).
261.Ebrahimi R, Faseleh Jahromi M, Liang JB, Soleimani Farjam A, Shokryazdan P and Idrus Z. Effect of dietary lead on intestinal nutrient transporters mRNA expression in broiler chickens. BioMed Res Int, 2015, 149745. doi: 10.1155/2015/149745.
262.Zhu Y, Lu X, Wu D, Cai S, Li S and Teng X. The effect of manganese-induced cytotoxicity on mRNA expressions of HSP27, HSP40, HSP60, HSP70 and HSP90 in chicken spleen lymphocytes in vitro. Biol Trace Elem Res, 156(1-3), 2013, 144-152.
263.Surai PF. Vitamin E in avian reproduction. Poult Avian Biol Rev, 10, 1999, 1-60.
264.Surai PF and Fisinin VI. Antioxidant Systems of the Body: From Vitamin E to Polyphenols and Beyond. Proceedings of the 35th Western Nutrition Conference, Edmonton, Alberta, 2014, 265-277.
265.El Golli E, Hassen W, Bouslimi A, Bouaziz C, Ladjimi MM and Bacha H. Induction of Hsp 70 in Vero cells in response to mycotoxins cytoprotection by sub-lethal heat shock and by Vitamin E. Toxicol Lett, 166(2), 2006, 122-130.
266.Soleimani AF, Zulkifli I, Omar AR and Raha AR. The relationship between adrenocortical function and Hsp70 expression in socially isolated Japanese quail. Comp Biochem Physiol A Mol Integr Physiol, 161(2), 2012, 140-144
267.Aggarwal A, Ashutosh Chandra G and Singh AK. Heat shock protein 70, oxidative stress, and antioxidant status in periparturient crossbred cows supplemented with α-tocopherol acetate. Trop Anim Health Prod, 45(1), 2013, 239-245.
268.Andrés D, Alvarez AM, Díez-Fernández C, Zaragoza A and Cascales M. HSP70 induction by cyclosporine A in cultured rat hepatocytes: effect of vitamin E succinate. J Hepatol, 33(4), 2000, 570-579.
269.Fischer CP, Hiscock NJ, Basu S, Vessby B, Kallner A, Sjöberg LB, Febbraio MA and Pedersen BK. Vitamin E isoformspecific inhibition of the exercise-induced heat shock protein 72 expression in humans. J Appl Physiol, 100(5), 2006, 1679-1687.
270.Reed DK, Hall S and Arany I. α-Tocopherol protects renal cells from nicotine- or oleic acid-provoked oxidative stress via inducing heme oxygenase-1. J Physiol Biochem, 71, 2015, 1-7.
271.Bellezza I, Tucci A, Galli F, Grottelli S, Mierla AL, Pilolli F and Minelli A. Inhibition of NF-κB nuclear translocation via HO-1 activation underlies α-tocopheryl succinate toxicity. J Nutr Biochem, 23, 2012, 1583-1591.
272.Feng Z, Liu Z, Li X, Jia H, Sun L, Tian C, Jia L and Liu J. α-Tocopherol is an effective Phase II enzyme inducer: protective effects on acrolein-induced oxidative stress and mitochondrial dysfunction in human retinal pigment epithelial cells. J Nutr Biochem, 21, 2010, 1222-1231.
273.Barve A, Khor TO, Nair S, Reuhl K, Suh N, Reddy B, Newmark H and Kong AN. Gamma-tocopherol-enriched mixed tocopherol diet inhibits prostate carcinogenesis in TRAMP mice. Int J Cancer, 124, 2009, 1693-1699.
274.Zhang B, Tanaka J, Yang L, Yang L, Sakanaka M, Hata R, Maeda N and Mitsuda N. Protective effect of vitamin E against focal brain ischemia and neuronal death through induction of target genes of hypoxia-inducible factor-1. Neuroscience, 126, 433-440.
275.Chakraborthy A, Ramani P, Sherlin HJ, Premkumar P and Natesan A. Antioxidant and pro-oxidant activity of Vitamin C in oral environment. Indian J Dent Res, 25(4), 2014, 499-504.
276.Mahmoud KZ, Edens FW, Eisen EJ and Havenstein GB. Ascorbic acid decreases heat shock protein 70 and plasma corticosterone response in broilers (Gallus gallus domesticus) subjected to cyclic heat. Comp Biochem Physiol B Biochem Mol Biol, 137, 2004, 35–42.
277.Mahmoud KZ, Edens FW, Eisen EJ and Havenstein GB. Effect of ascorbic acid and acute heat exposure on heat shock protein 70 expression by young white Leghorn chickens. Comp Biochem Physiol C Toxicol Pharmacol, 136(4), 2003, 329-335.
278.Sahin N, Tuzcu M, Orhan C, Onderci M, Eroksuz Y and Sahin K. The effects of vitamin C and E supplementation on heat shock protein 70 response of ovary and brain in heat-stressed quail. Br Poult Sci, 50(2), 2009, 259-265.
279.Khassaf M, McArdle A, Esanu C, Vasilaki A, McArdle F, Griffiths RD, Brodie DA and Jackson MJ. Effect of vitamin C supplements on antioxidant defence and stress proteins in human lymphocytes and skeletal muscle. J Physiol, 549(Pt 2), 2003, 645-652.
280.Simar D, Malatesta D, Mas E, Delage M and Caillaud C. Effect of an 8-weeks aerobic training program in elderly on oxidative stress and HSP72 expression in leukocytes during antioxidant supplementation. J Nutr Health Aging, 16(2), 2012, 155-161.
281.Sánchez-Moreno C, Paniagua M, Madrid A and Martín A. Protective effect of vitamin C against the ethanol mediated toxic effects on human brain glial cells. J Nutr Biochem, 14(10), 2003, 606-613.
282.Ji YL, Wang Z, Wang H, Zhang C, Zhang Y, Zhao M, Chen YH, Meng XH and Xu DX. Ascorbic acid protects against cadmium-induced endoplasmic reticulum stress and germ cell apoptosis in testes. Reprod Toxicol, 34(3), 2012, 357-363.
283.Yun SH, Moon YS, Sohn SH and Jang IS. Effects of cyclic heat stress or vitamin C supplementation during cyclic heat stress on HSP70, inflammatory cytokines, and the antioxidant defense system in Sprague Dawley rats. Exp Anim, 61(5), 2012, 543-553.
284.Yazama F, Furuta K, Fujimoto M, Sonoda T, Shigetomi H, Horiuchi T, Yamada M, Nagao N and Maeda N. Abnormal spermatogenesis in mice unable to synthesize ascorbic acid. Anat Sci Int, 81(2), 2006, 115-125.
285. Zhao B, Fei J, Chen Y, Yin Y-L, Ma L, Song X-Q, Huang J, Chen E-Z and Mao E-Q. Vitamin C treatment attenuates hemorrhagic shock related multi-organ injuries through the induction of heme oxygenase-1. BMC Complement Altern Med, 14, 2014, 442.
286.Zhong W, Gu B, Gu Y, Groome LJ, Sun J and Wang Y. Activation of vitamin D receptor promotes VEGF and CuZnSOD expression in endothelial cells. J Steroid Biochem Mol Biol, 140, 2014, 56–62.
287.Xu S, Chen YH, Tan ZX, Xie DD, Zhang C, Xia MZ, Wang H, Zhao H, Xu DX and Yu DX. 2015. Vitamin D3 pretreatment alleviates renal oxidative stress in lipopolysaccharide-induced acute kidney injury. J Steroid Biochem Mol Biol, 152, 2015, 133-141.
288.Zhu L, Kong M, Han YP, Bai L, Zhang X, Chen Y, Zheng S, Yuan H and Duan Z. Spontaneous liver fibrosis induced by long term dietary vitamin D deficiency in adult mice is related to chronic inflammation and enhanced apoptosis. Can J Physiol Pharmacol, 93, 2015, 385-394.
289. Roth CL, Elfers CT, Figlewicz DP, Melhorn SJ, Morton GJ, Hoofnagle A, Yeh MM, Nelson JE and Kowdley KV. 2012. Vitamin D deficiency in obese rats exacerbates NAFLD and increases hepatic resistin and toll-like receptor activation. Hepatology, 55, 2012, 1103-1111.
290.Shih PK, Chen YC, Huang YC, Chang YT, Chen JX and Cheng CM. Pretreatment of vitamin D3 ameliorates lung and muscle injury induced by reperfusion of bilateral femoral vessels in a rat model. J Surg Res, 171, 2011, 323–328.
291.Kutuzova GD and DeLuca HF. 1,25-Dihydroxyvitamin D3 regulates genes responsible for detoxification in intestine. Toxicology and Applied Pharmacology, 218, 2007, 37-44.
292.Oermann E, Bidmon HJ, Witte OW, Zilles K. Effects of 1alpha,25dihydroxyvitamin D3 on the expression of HO-1 and GFAP in glial cells of the photothrombotically lesioned cerebral cortex. J Chem Neuroanat, 28, 2004. 225-238.
293.Alirezaei M, Khoshdel Z, Dezfoulian O, Rashidipour M, Taghadosi V. Beneficial antioxidant properties of betaine against oxidative stress mediated by levodopa/benserazide in the brain of rats. J Physiol Sci, 65(3), 2015, 243-252.
294.Calò LA, Pagnin E, Davis PA, Semplicini A, Nicolai R, Calvani M and Pessina AC. Antioxidant effect of L-carnitine and its short chain esters: relevance for the protection from oxidative stress related cardiovascular damage. Int J Cardiol, 107, 2006, 54-60.
295.Calò LA, Davis PA, Pagnin E, Bertipaglia L, Naso A, Piccoli A, Corradini R, Spinello M, Savica V and Dalla Libera L. Carnitine-mediated improved response to erythropoietin involves induction of haem oxygenase-1: studies in humans and in an animal model. Nephrol Dial Transplant, 23, 2008, 890-895.
296.Cao Y, Li X, Wang CJ. Li P, Yang B, Wang CB and Wang LX. Role of NF-E2-relatedfactor 2 in neuroprotective effect of l-carnitine against high glucose-induced oxidative stress in the retinal ganglion cells. Biomed Pharmacother, 69, 2015, 345-348.
297.Selim ME, Rashed el HA, Aleisa NA and Daghestani MH. The protection role of heat shock protein 70 (HSP-70) in the testes of cadmium-exposed rats. Bioinformation, 8, 2012, 58-64.
298.Abdul HM, Calabrese V, Calvani M and Butterfield DA. Acetyl-L-carnitine-induced up-regulation of heat shock proteins protects cortical neurons against amyloid-beta peptide 1-42-mediated oxidative stress and neurotoxicity: implications for Alzheimer's disease. J Neurosci Res, 84, 2006, 398-408.
299.Calabrese V, Ravagna A, Colombrita C, Scapagnini G, Guagliano E, Calvani M, Butterfield DA, Giuffrida Stella AM. Acetylcarnitine induces heme oxygenase in rat astrocytes and protects against oxidative stress: involvement of the transcription factor Nrf2. J Neurosci Res, 79, 2005, 509–521.
300.Lordnejad MR, Schliess F, Wettstein M and Häussinger D. Modulation of the hemeoxygenase HO-1 expression by hyperosmolarity and betaine in primary rat hepatocytes. Arch Biochem Biophys, 388, 2001, 285-292.
301.Chen X, Zhu YH, Cheng XY, Zhang ZW and Xu SW. The protection of selenium against cadmium-induced cytotoxicity via the heat shock protein pathway in chicken splenic lymphocytes. Molecules, 17(12), 2012, 14565-14572.
302.Xu Z, Wang Z, Li JJ, Chen C, Zhang PC, Dong L, Chen JH, Chen Q, Zhang XT and Wang ZL. Protective effects of selenium on oxidative damage and oxidative stress related gene expression in rat liver under chronic poisoning of arsenic. Food Chem Toxicol, 58, 2013, 1-7.
303.Mahmoud KZ and Edens FW. Influence of organic selenium on hsp70 response of heat-stressed and enteropathogenic Escherichia coli-challenged broiler chickens (Gallus gallus). Comp Biochem Physiol C Toxicol Pharmacol, 141(1), 2005, 69-75.
304.Mahmoud KZ and Edens FW. Influence of selenium sources on age-related and mild heat stress-related changes of blood and liver glutathione redox cycle in broiler chickens (Gallus domesticus). Comp Biochem Physiol B Biochem Mol Biol, 136(4), 2003, 921-934.
305.Rivera RE, Christensen VL, Edens FW and Wineland MJ. Influence of selenium on heat shock protein 70 expression in heat stressed turkey embryos (Meleagris gallopavo). Comp Biochem Physiol A Mol Integr Physiol, 142(4), 2005, 427- 432.
306.Xu D, Li W, Huang Y, He J and Tian Y. The effect of selenium and polysaccharide of Atractylodes macrocephala Koidz. (PAMK) on immune response in chicken spleen under heat stress. Biol Trace Elem Res, 160(2), 2014, 232-237.
307.Gan F, Ren F, Chen X, Lv C, Pan C, Ye G, Shi J, Shi X, Zhou H, Shituleni SA and Huang K. Effects of seleniumenriched probiotics on heat shock protein mRNA levels in piglet under heat stress conditions. J Agric Food Chem, 61(10), 2013, 2385-2391.
308.Dangi SS, Gupta M, Dangi SK, Chouhan VS, Maurya VP, Kumar P, Singh G and Sarkar M. Expression of HSPs: an adaptive mechanism during long-term heat stress in goats (Capra hircus). Int J Biometeorol, 59(8), 2015, 1095-1106.
309.Chen X., Yao H., Yao L., Zhao J., Luan Y., Zhang Z., Xu S., 2014. Selenium deficiency influences the gene expressions of heat shock proteins and nitric oxide levels in neutrophils of broilers. Biol Trace Elem Res, 161(3), 334-340.
310.Liu CP, Fu J, Xu FP, Wang XS and Li S. The role of heat shock proteins in oxidative stress damage induced by Se deficiency in chicken livers. Biometals, 28(1), 2015, 163-173.
311.Yang Z, Liu C, Zheng W, Teng X and Li S. The Functions of Antioxidants and Heat Shock Proteins Are Altered in the Immune Organs of Selenium-Deficient Broiler Chickens. Biol Trace Elem Res, 2015. (In Press, Epub ahead of print).
312.Khoso PA, Yang Z, Liu C and Li S. Selenoproteins and heat shock proteins play important roles in immunosuppression in the bursa of Fabricius of chickens with selenium deficiency. Cell Stress Chaperones, 2015. (In Press, Epub ahead of print).
313.Kaur P and Bansal MP. Effect of oxidative stress on the spermatogenic process and hsp70 expressions in mice testes. Indian J Biochem Biophys, 40(4), 2003, 246-251.
314.Kaushal N and Bansal MP. Diminished reproductive potential of male mice in response to selenium-induced oxidative stress: involvement of HSP70, HSP70-2, and MSJ-1. J Biochem Mol Toxicol, 23(2), 2009, 125-136.
315.Zhu X, Guo K, Lu Y. Selenium effectively inhibits 1,2-dihydroxynaphthalene-induced apoptosis in human lens epithelial cells through activation of PI3-K/Akt pathway. Mol Vis, 17, 2011, 2019-2027.
316.Yousuf S, Atif F, Ahmad M, Hoda MN, Khan MB, Ishrat T and Islam F. Selenium plays a modulatory role against cerebral ischemia-induced neuronal damage in rat hippocampus. Brain Res, 1147, 2007, 218-225.
317.de Roos B and Duthie GG. Role of dietary pro-oxidants in the maintenance of health and resilience to oxidative stress. Mol Nutr Food Res, 59(7), 2015, 1229-1248.
318.Murakami A. Modulation of protein quality control systems by food phytochemicals. J Clin Biochem Nutr, 52(3), 2013, 215-227.
319.Calabrese V, Cornelius C, Dinkova-Kostova AT, Iavicoli I, Di Paola R, Koverech A, Cuzzocrea S, Rizzarelli E, Calabrese EJ. Cellular stress responses, hermetic phytochemicals and vitagenes in aging and longevity. Biochim Biophys Acta, 1822(5), 2011, 753-783.
320.Mattson MP and Cheng A. Neurohormetic phytochemicals: Low-dose toxins that induce adaptive neuronal stress responses. Trends Neurosci, 29(11), 2006, 632-639.
321.Muthumani M and Prabu SM. Silibinin potentially attenuates arsenic-induced oxidative stress mediated cardiotoxicity and dyslipidemia in rats. Cardiovasc Toxicol, 14, 2014, 83–97.
322.Choi MK, Han JM, Kim HG, Lee JS, Lee JS, Wang JH, Son SW, Park HJ and Son CG. Aqueous extract of Artemisia capillaries exerts hepatoprotective action in alcohol-pyrazole-fed rat model. J Ethnopharmacol, 147, 2013, 662–670.
323.Kiruthiga PV, Karthikeyan K, Archunan G, Karutha Pandian S and Pandima Devi K. Silymarin prevents benzo(a)pyrene-induced toxicity in Wistar rats by modulating xenobiotic-metabolizing enzymes. Toxicol Ind Health, 31(6), 2015, 523-541.
324.Cerný D, Canová NK, Martínek J, Horínek A, Kmonícková E, Zídek Z and Farghali H. Effects of resveratrol pretreatment on tert-butylhydroperoxide induced hepatocyte toxicity in immobilized perifused hepatocytes: Involvement of inducible nitric oxide synthase and hemoxygenase-1. Nitric Oxide, 20, 2009, 1–8.
325.Venditti CC and Smith GN. Involvement of the heme oxygenase system in the development of preeclampsia and as a possible therapeutic target. Women’s Health (London, England), 10, 2014, 623–643.
326.Bongiovanni GA, Soria EA and Eynard AR. Effects of the plant flavonoids silymarin and quercetin on arsenite-induced oxidative stress in CHO-K1 cells. Food Chem Toxicol, 45, 2007, 971–976.
327.Oskoueian E, Abdullah N, Idrus Z, Ebrahimi M, Goh YM, Shakeri M and Oskoueian A. Palm kernel cake extract exerts hepatoprotective activity in heat-induced oxidative stress in chicken hepatocytes. BMC Complement Altern Med, 14, 2014, 368.
328.Demir M, Amanvermez R, Kamali Polat A, Karabiçak I, Cinar H, Kesicioglu T and Polat C. The effect of silymarin on mesenteric ischemia-reperfusion injury. Med Princ Prac, 23, 2014, 140–144.
329.Zhao H, Brandt GE, Galam L, Matts RL and Blagg BS. Identification and initial SAR of silybin: An Hsp90 inhibitor. Bioorg Med Chem Lett, 21, 2011, 2659–2664.
330.Liu LL, He JH, Xie HB, Yang YS, Li JC and Zou Y. 2014. Resveratrol induces antioxidant and heat shock protein mRNA expression in response to heat stress in black-boned chickens. Poult Sci, 93(1), 2014, 54-62.
331.Surai PF and Fisinin VI. The modern anti-stress technologies in poultry: from antioxidants to vitagenes. Agricultural Biology (Sel’skokhozyaistvennaya Biologiya, Moscow), 4, 2012, 3-13.
332.Fotina A, Fotina TI and Surai P.F. Effect of a water-soluble antistress composition on broiler chickens”. In Proceedings of the XIVth European Poultry Conference, Stavanger, Norway, 2014, 555.
333.Velichko O and Surai PF. Effect of an antistress composition supplied with water on chick growth and development”. In Proceedings of the XIVth European Poultry Conference, Stavanger, Norway, 2014, 551.
334.Gupta SC, Sharma A, Mishra M, Mishra RK and Chowdhuri DK. Heat shock proteins in toxicology: how close and how far? Life Sci, 86(11-12), 2010, 377-384.
335.Bozaykut P, Ozer NK and Karademir B. Regulation of protein turnover by heat shock proteins. Free Radic Biol Med, 77, 2014, 195-209.
336.O'Neill S, Harrison EM, Ross JA, Wigmore SJ and Hughes J. Heat-shock proteins and acute ischaemic kidney injury. Nephron Exp Nephrol, 126(4), 2014, 167-174.
337.Khalil AA, Kabapy NF, Deraz SF and Smith C. Heat shock proteins in oncology: diagnostic biomarkers or therapeutic targets? Biochim Biophys Acta, 1816(2), 2011, 89-104.