Introduction
Heat stress (HS) is a major environmental hazard for both humans and animals. Heat claims more human lives than all other climatic events combined (Changnon et al. 1996), with the young and elderly populations being the most susceptible (Leon and Helwig 2010). Surprisingly, despite increased understanding on the pathophysiology of heat-related illnesses (Bouchama and Knochel 2002), the only standard procedures to treat heat victims are cooling and rehydration (Leon and Helwig 2010).
In addition to morbidity and mortality, HS negatively impacts livestock productivity. Environmental hyperthermia costs global animal agriculture several billion dollars annually due to reduced and inconsistent growth, decreased carcass quality, compromised reproduction, reduced milk yield and egg production, and increased veterinary costs (St-Pierre et al. 2003; Baumgard and Rhoads 2013). We have previously reported that, despite hypercatabolic hallmarks like marked hypophagia and weight loss, HS increases basal and stimulated (i.e., in response to a metabolic challenge) circulating insulin and decreases adipose tissue mobilization in a variety of species (Baumgard and Rhoads 2013), including pigs (Pearce et al. 2013a). Interestingly, diabetic humans and rodents are more susceptible to heat-related illnesses and exogenous insulin rescues this phenotype (Semenza et al. 1999; Niu et al. 2003). Furthermore, thermal therapy improves insulin sensitivity in diabetic and obese rodents (Kokura et al. 2007; Gupte et al. 2009) and humans (Hooper 1999). Collectively, these reports suggest that insulin or altered insulin action and subsequent shifts in metabolism are a conserved response among species that might play an important role in the adaptation and survivability to HS (Baumgard and Rhoads 2013).
Thus, the first study objective was to determine the effects of HS on the temporal responses to acute metabolic challenges in pigs. Our second objective was to investigate the systemic and molecular changes responsible for the HS-induced shift in postabsorptive metabolism. We hypothesized that HS has a direct effect on metabolism, independent of the heat-induced hypophagia. The pig is both a relevant biomedical model (Prather et al. 2013) and an important agricultural species, thus results will likely have important implications in both human health and animal production.
Materials and Methods
Animals and experimental design
Iowa State University Institutional Animal Care and Use Committee approved all procedures involving animals. Seventeen crossbred female pigs (57 ± 5 kg body weight) were randomly assigned to 1 of 2 treatments during two experimental periods. Prepubertal female pigs were utilized to be consistent with our previous experiments (Sanz-Fernandez et al. 2012; Pearce et al. 2013a,b; Sanz Fernandez et al. 2014). During period 1 (4 days in length), all pigs were exposed to thermoneutral conditions [TN; 20°C, ~35% humidity (Federation of Animal Sciences Societies 2010)] and fed ad libitum. During period 2 (8 days in length), pigs were either exposed to constant HS conditions (32°C, ~23% humidity (Federation of Animal Sciences Societies 2010); n = 7) and fed ad libitum or remained in TN conditions and were pair-fed (PFTN; n = 10) to their HS counterparts, in order to eliminate the confounding effect of dissimilar nutrient intake. For the pair-feeding calculations, as-fed period 1 daily feed intake (FI) was averaged for each pig and used as a baseline. For each HS pig, the decrease in intake during period 2 was calculated as the percentage of FI reduction relative to period 1 for each day of HS exposure. This percentage of FI reduction was averaged for all the HS pigs per day of exposure and applied individually to the baseline of each PFTN pig. The calculated amount of feed was offered to the PFTN pigs three times a day (~0800, 1400, and 2100 h) in an attempt to minimize large postprandial shifts in metabolism. All pigs were fed a standard swine grower diet consisting mainly of corn and soybean meal formulated to meet or exceed nutrient requirements (National Research Council 2012). Pigs were individually housed in metabolic crates in 1 of 6 environmental chambers where temperature was controlled but humidity was not governed. Both parameters were recorded every 30 min by a data logger (Lascar EL-USB-2-LCD, Erie, PA). Rectal temperature was measured with a digital thermometer (ReliOn, Waukega, IL), respiration rate was determined by counting flank movements, and both indices were measured twice a day (0700 and 2200 h) and condensed into daily averages. Pigs were killed on d 8 of period 2 using the captive bolt technique and subcutaneous adipose tissue (AT) from the cranial dorsum, skeletal muscle from longissimus dorsi (LD), liver, and pancreas samples were immediately collected and snap frozen or preserved for histology.
Blood sampling and metabolic challenges
An indwelling jugular catheter was surgically inserted on d 1 of period 1 in all pigs. Pigs were anesthetized with a mixture of tiletamine/zolazepam (Telazol; Fort Dodge Laboratories Inc., Fort Dodge, IA), ketamine (Ketaject; Clipper Distributing Company, LLC, St Joseph, MO), and xylazine (Anased; Lloyd Inc., Shenandoah, IA) for a final concentration of 100, 50, and 50 mg/mL, respectively. Pigs were injected IM with the anesthetics mixture at 1 mL/23 kg BW dose. With the pigs in dorsal recumbency, the jugular vein was located with a 14 G, 3.75 cm introducer needle (Mila International Inc., Erlanger, KY) using a percutaneous technique. Once in the vein, Tygon tubing (1.016 mm inside diameter, 1.778 mm outside diameter; Saint-Gobain Performance Plastic Corp., Aurora, OH) was inserted through the introducer needle (15 cm). After removing the introducer, a small skin incision was made cranial to the tube’s insertion point exposing the subcutaneous tissue. Using laparoscopic forceps (Auto Suture Endo GraspTM 5 mm; Covidien, Mansfield, MA), the tube was tunneled subcutaneously from the incision and exteriorized at the dorsum of the back. The neck incision was sutured and the catheter remained subcutaneous and not exposed. All pigs received antibiotics (Ceftiofur, Excede; Pfizer Animal Health, New York, NY) and nonsteroidal antiinflammatory drugs (Flunixin meglumine, Banamine-S; ScheringPlough Animal Health Corp., Whitehouse Station, NJ) during the surgery. Intravenous antibiotic therapy continued daily for the rest of the study (Ampicillin, Poly- flex; Fort Dodge Laboratories Inc., Fort Dodge, IA) and catheters were flushed twice daily with heparinized saline (100 U/mL).
Animals were fasted for 2 h prior to the metabolic challenges and daily blood sampling (basal metabolism samples). Glucose tolerance tests (GTT) were performed on d 3 of period 1 and d 1 and 6 of period 2. A 50% dextrose solution bolus (VetOne; MWI Veterinary Supply, Boise, ID) was administered at 0.5 g/kg BW dose via the jugular catheter. Blood samples were collected at 20, 10, 0, 5, 7.5, 10, 15, 20, 30, 45, 60 min relative to the glucose administration. Epinephrine challenges (EC; 3 lg/ kg BW; American Regent, Inc., Shirley, NY) were performed on d 4 of period 1 and d 2 and 7 of period 2 and blood samples were collected at 20, 10, 0, 2.5, 5, 7.5, 10, 15, 20, 30, 45, 60 min relative to the epinephrine administration. Daily blood samples were obtained at 0800 h so animals in both treatments were in a preprandial state. All blood samples were collected into disposable glass tubes containing 250 U of sodium heparin and immediately placed on ice. Plasma was harvested by centrifugation at 1300 x g for 15 min at 4°C and stored at 80°C for later analysis.
Blood parameters analyses
Plasma glucose and nonesterified fatty acids (NEFA) concentrations were measured enzymatically using commercially available kits (Wako Chemicals USA, Richmond, VA). The intra and interassay coefficients of variation were 4.3 and 3.6%, and 7.3 and 5.8% for glucose and NEFA, respectively. Plasma β-hydroxybutyrate (BHB) concentration was also analyzed enzymatically using a commercially available kit (Pointe Scientific Inc., Canton, MI) and the intra and interassay coefficients of variation were 3.2 and 2.2%. Plasma insulin and C-peptide concentrations were analyzed using ELISA kits (Mercodia AB, Uppsala, Sweden) following the manufacturer’s instructions. The intra and interassay coefficients of variation were 2.9 and 6.3%, and 7.2 and 4.9% for insulin and Cpeptide, respectively. Plasma lipopolysaccharide (LPS)- binding protein (LBP) concentration was determined using an ELISA kit (Hycult biotech, Uden, The Netherlands) and the intraassay coefficients of variation was 1.5%. Total plasma thyroxine (T4) and triiodothyronine (T3) concentrations were evaluated using commercially available solid phase RIA kits (MP Biomedicals, LLC; Irvine, CA) according to the manufacturer’s instructions. The assay kits were validated (recovery and linearity of diluted samples) for use with porcine plasma samples as previously described (Kahl et al. 2000), and the intraassay coefficients of variation were 3.7 and 4.1% for T3 and T4, respectively.
Hepatic 5'-deiodinase type I (5'D) activity
Outer-ring deiodinating activity was determined by quantifying the 125I- source released from 3,3' ,5' -[125I]-T3 (rT3) as previously described (Kahl et al. 2000). In brief, hepatic samples were homogenized in 0.01 mol/L HEPES buffer (pH 7.0, 0.25 mol/L sucrose, 5 mmol/L EDTA). After centrifugation (30 min at 2000 x g), the supernatant was incubated for 5 min in 0.1 mol/L phosphate buffer (pH 7.0, 1 mmol/L EDTA) in the presence of 5 mmol/L dithiothreitol at 37°C with approximately 80,000 cpm of [ 125I]-rT3 (DuPont-New England Nuclear, Boston, MA) and 500 nmol/L of unlabeled rT3 (Calbiochem, La Jolla, CA). The released 125I- source was isolated as trichloroacetic acid-soluble radioactivity. The 5'D activity was expressed as nmol of I- produced per mg protein per hour. Protein concentration in homogenates was determined by bicinchoninic acid assay (BCA, PierceTM; Thermo Fisher Scientific Inc., Rockford, IL). The intraassay coefficient of variation was 8.6%.
Pancreatic insulin content
Pancreatic protein was extracted at 4°C overnight with acid-ethanol (75% ethanol, 1.5% HCl). After centrifugation at 2000 rpm for 15 min, the supernatant was neutralized with 1 mol/L Tris (pH = 7.5) and total protein was determined by BCA assay (PierceTM; Thermo Fisher Scientific Inc., Rockford, IL). Neutralized samples were further diluted (1:20000) and insulin concentration was measured using an ELISA kit (Mercodia AB, Uppsala, Sweden) following the manufacturer’s instructions. Pancreatic insulin content was calculated relative to the total amount of protein.
Pancreatic immunohistochemistry
Pancreatic tissue samples were fixed in 10% formalin for 24 h and then transferred into 70% ethanol. Fixed samples were sectioned at 4 μm thickness. Two random sections per pig were stained for insulin using an indirect immunoperoxidase technique. The primary and secondary antibodies (Dako, Carpinteria, CA) were a polyclonal guinea pig antiporcine insulin antibody (Dilution 1:1000) and a polyclonal rabbit antiguinea pig horse radish peroxidase-conjugated immunoglobulin (Dilution 1:50), respectively. Diaminobenzidine (Dako, Carpinteria, CA) was used as the chromogen and Mayer’s hematoxylin as the counterstain. Negative controls were generated by omitting the primary or the secondary antibody. Five nonoverlapping fields per section were imaged at 50 x using Qcapture Pro 6.0 software (Qimaging, Surey, BC) with the operator being blind to the treatments. The relativestained area was measured by thresholding and the number and size of insulin-stained cell clusters were determined using the particle analysis tool in ImageJ (US National Institutes of Health, Bethesda, MD). The results obtained in each section were averaged into a single measurement per pig.
RNA isolation and quantitative PCR
Total RNA was extracted from AT, LD and liver using TRIzol Reagent (Invitrogen, Carlsbad, CA) following the manufacturer’s protocol and was utilized for cDNA synthesis using the QuantiTect Reverse Transcription Kit (Qiagen, Hilden, Germany). Gene expression differences were determined using qPCR (BioMarkTM System, Fluidigm Corporation, San Francisco, CA) on 40, 44, and 44 genes for AT, LD, and liver, respectively (Tables 3–5). Genes chosen for analysis were selected based on the RNA-Seq output of a similar experiment (Seibert et al. 2014). Expression normalization across samples within a tissue was performed by calculating a delta Ct (ΔCt) value for each sample using GAPDH for AT, β-actin for LD, and RPL32 in liver, as transcript abundance for these genes proved to be the most similar between treatments for each given tissue (P > 0.05). ΔΔCt values were calculated utilizing a reference sample and fold differences between treatments were obtained by applying the equation 2|ΔΔCt PFTN-ΔΔCt HS|, where a positive and a negative value indicate an increase and a decrease in transcript abundance, respectively, in HS pigs relative to PFTN controls. Statistical analysis was performed on the ΔΔCt values and data are reported in the results section as relative fold difference.
Calculations and statistical analysis
Metabolic responses to the GTT and EC were calculated as area under the curve (AUC) by linear trapezoidal summation between successive pairs of metabolite concentrations and time coordinates after subtracting baseline values. Glucose and insulin AUC in response to the GTT were calculated through min 20 of the challenge. Glucose and NEFA responses to the EC were determine through min 30 and 15, respectively. For the GTT, glucose and insulin deltas were calculated for each challenge as the change in their concentration between 0 and 5 min, and 0 and 7.5 min, respectively. In addition, glucose disappearance was calculated as the slope of glucose concentrations between 5 and 30 min. An insulinogenic index was determined as the insulin AUC to glucose AUC ratio.
All data were statistically analyzed using SAS version 9.3 (SAS Institute Inc., Cary, NC). Single measurements were analyzed using PROC MIXED and the model included treatment as fixed effect. Variables with multiple measurements per pig over time were analyzed using PROC MIXED with day of period 2 as the repeated effect and period 1 values used as a covariate. Auto regressive and spatial power covariance structures were utilized for equally and unequally spaced measurements, respectively. The model included treatment, day and their interaction as fixed effects. For each variable, normal distribution of residuals was tested using PROC UNIVARIATE and logarithmic transformation was performed when necessary. Data are reported as least square means and considered significant if P ≤ 0.05 and a tendency if 0.05 < P ≤ 0.10.
Results
As expected, during period 2 HS pigs had increased rectal temperature and respiration rate (1.5°C and 4.5 fold, respectively; P < 0.01) compared to PFTN controls (Table 1). Heat stress decreased FI (39%, P < 0.01), and by design, PFTN pigs’ FI was reduced similarly (Table 1). Overall, this data indicate that pigs experienced an acute and sustained heat load indicative of marked heat stress.
During period 2, basal glucose concentrations decreased progressively with time (P < 0.01) in both treatments; however, HS pigs became overall more hypoglycemic (6%, P = 0.02) than PFTN pigs (Fig. 1A). No treatment differences were detected on basal plasma insulin (Fig. 1B) or the insulin to glucose ratio (Fig. 1C), but there was a treatment by day interaction on plasma C-peptide and Cpeptide to glucose ratio (P = 0.01) as they were increased on d 1 (48 and 44%, respectively) and 3 (61 and 64%, respectively) in HS pigs compared to PFTN controls, but no treatment differences were detected on d 7 (Fig. 1D and E). During period 2, basal plasma NEFA acutely increased on d 1 and progressively decreased thereafter (P < 0.01) in both treatments, but overall HS pigs tended to have reduced basal NEFA (20%, P = 0.07) than PFTN pigs (Fig. 1F). There was a tendency for a treatment by day interaction on plasma BHB (P = 0.10), as HS pigs had increased BHB levels on d 1 (44%) compared to PFTN pigs, but similar BHB concentrations thereafter (Fig. 1G). Circulating LBP decreased with time (P = 0.05) and there was a tendency for a treatment by day interaction (P = 0.08), as HS pigs had numerically increased LBP concentrations on d 1 (20%) and d 3 (56%) compared with PFTN controls, but plasma LBP did not differ between treatments on d 7 (Fig. 1H). At the initiation of period 2, plasma T3 concentrations decreased, but progressively increased over time (P < 0.01) for both treatments (Fig. 2A). However, the d 1 decrease was more severe in HS pigs (68%) and this difference was maintained through the end of period 2 (P < 0.01; Fig. 2A). There was a treatment by day interaction on plasma T4 (P < 0.01) as HS pigs had decreased concentrations compared to PFTN pigs on d 1 and 3 (36 and 40%, respectively), but circulating T4 did not differ between treatments on d 7 (Fig. 2B). Overall, HS pigs had decreased T3 to T4 ratio (56%, P = 0.01) compared to PFTN controls (Fig. 2C). After 8 days of environmental treatment, hepatic 50 D activity was reduced (58%; P < 0.01) in HS pigs compared to PFTN controls (Fig. 2D).
Table 1. Effects of pair-feeding or heat stress on body temperature indices and feed intake.
Figure 1. Effects of ad libitum feed intake in constant heat stress conditions (HS; 32°C) and pair-feeding in thermoneutral conditions (PFTN; 20°C) on temporal changes in plasma (A) glucose, (B) insulin, (C) insulin to glucose ratio (Insulin:G) (D) C-peptide, (E) C-peptide to glucose ratio (C-peptide:G), (F) nonesterified fatty acids (NEFA), (G) b-hydroxybutyrate (BHB), and (H) lipopolysaccharide-binding protein (LBP). Values on day 0 represent period 1 average that was statistically used as covariate. a-dMeans with different superscript differ (P ≤ 0.05). x-zDays with different superscript differ (P ≤ 0.05).
Figure 2. Effects of ad libitum feed intake in constant heat stress conditions (HS; 32°C) and pair-feeding in thermoneutral conditions (PFTN; 20°C) on temporal changes in plasma (A) T3, (B) T4, (C) T3 to T4 ratio (T3:T4); and (D) hepatic 5’-deiodinase type I activity (5’D) after 8 days of environmental treatment. Values on day 0 represent period 1 average that was statistically used as covariate. a–c Means with different superscript differ (P ≤ 0.05).
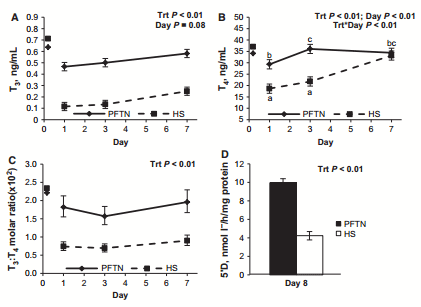
During period 2 there was a treatment by day interaction on the glucose response to the GTT (P < 0.01) as HS pigs had an increased glucose AUC (15%) on d 1, but not on d 6 (Table 2). There was a treatment by day interaction on the glucose delta in response to the GTT (P = 0.04), as it did not change from day 1 to 6 in PFTN pigs but decreased (10%) in HS pigs (Table 2). Glucose disappearance following glucose administration tended to increase (13%; P = 0.06) from day 1 to 6, however, overall, it tended to be decreased (14%; P = 0.07) in HS pigs compared to PFTN counterparts (Table 2). There was a tendency for a treatment by day interaction on the insulin response to the GTT (P = 0.06) as HS pigs had a decreased (30%) insulin AUC on days 1 compared to PFTN pigs, but the insulin response was similar between treatments on d 6 (Table 2). Overall, the insulin delta in response to the GTT tended to be reduced (16%, P = 0.06) in HS pigs compared to PFTN controls (Table 2). There was a treatment by day interaction on the insulinogenic index (P < 0.01) as it was decreased (45%) in HS pigs on day 1 compared to PFTN controls, but did not differ between treatments on d 6 (Table 2).
Table 2. Effects of pair-feeding or heat stress on the response to metabolic challenges.
During period 2, the NEFA AUC in response to the EC decreased (43%; P < 0.01) from days 2 to 7, and overall it was reduced (71%; P = 0.01) in HS pigs compared to PFTN controls (Table 2). In contrast, the glucose response to the EC did not differ (P > 0.10) between treatments or change with time (Table 2).
After 8 days of environmental exposure, pancreatic insulin content did not differ (P > 0.17) between treatments (Fig. 3C). Based on the immunohistochemistry data, HS tended to decrease pancreatic insulin-stained area (32%; P = 0.08) compared to PFTN conditions (Fig. 3A, B, D). In addition, there were no treatment differences detected in the quantity of insulin-positive cell clusters (Fig. 3E). However, when classified by size, HS decreased (66%; P < 0.01) the percentage of larger clusters (diameter >100 lm), whereas increasing (4%; P ≤ 0.01) the percentage of smaller clusters (diameter <50 lm; Fig. 3F).
Figure 3. Effects of 8 days of ad libitum feed intake in constant heat stress conditions (HS; 32°C) and pair-feeding in thermoneutral conditions (PFTN; 20°C) pancreatic insulin content. (A) Representative picture of PFTN pancreas; (B) representative picture of PFTN pancreas; (C) insulin content; (D) insulin-stained area; (E) number of insulin-stained clusters; (F) insulin-stained clusters size distribution. *Represents differences with PFTN controls (P ≤ 0.05).
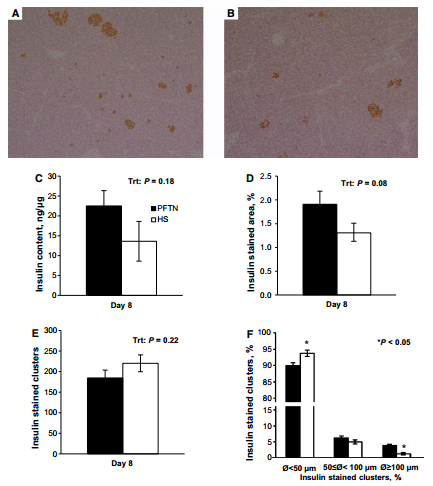
After 8 days of environmental treatment, HS increased or tended to increase transcript abundance for heat-shock proteins compared to PFTN conditions: HSPA4 (2.43; P = 0.08), HSPB8 (2.51; P = 0.10), and HSPE1 (2.57; P = 0.09), in AT; HSP90AA1 (2.10; P < 0.01), HSPA4 (1.52; P = 0.05), HSPCB (1.72; P = 0.02), and HSPE1 (2.38; P = 0.02), in LD; and HSPE1 (1.62; P = 0.08), in liver (Tables 3–5). In AT, transcripts for the following genes were or tended to be less abundant in HS compared to PFTN pigs: ATGL (-1.56; P = 0.07), INSR (-1.46; P = 0.07), MIF (-1.60; P = 0.10), PDAP1 (-1.73; P = 0.07), PRKAG1 (-1.89; P = 0.05), and ZSCAN29 (-1.77; P = 0.05; Table 3). In LD, HS conditions increased transcript abundance for CD14 (1.68; P = 0.01), and reduced or tended to reduce it for CKMT2 (-2.03; P = 0.10), IDH2 (-1.77; P = 0.06), NDUFS7 (-2.01; P = 0.06), PDK4 (-2.53; P = 0.05), and SLC16A5 (-2.60; P = 0.03); compared to PFTN conditions (Table 4). In liver, mRNA transcripts for FASN (2.62; P < 0.01), KCTD6 (1.36; P = 0.08), and RBM7 (1.31; P = 0.06) were or tended to be more abundant; whereas, NDUFB7 (-1.28; P = 0.05) and NDUFS7 (-1.29; P = 0.10) were or tended to be less abundant in HS pigs compared to PFTN controls (Table 5).
Discussion
Heat stress jeopardizes human health and compromises animal agriculture productivity. Despite advances in the understanding of heat-related illnesses, there is no treatment against specific aspects of their pathophysiology, and protocols are limited to generic cooling and hydration (Leon and Helwig 2010). Physically modifying the microenvironment with heat stress abatement strategies (shade, fans, evaporative cooling) has markedly enhanced agricultural productivity, but facility improvements have failed to completely prevent the loss in animal efficiency during the warm summer months (Stowell et al. 2009). Therefore, a better understanding of the biological consequences of HS is a prerequisite to developing treatment protocols and mitigation strategies targeting the physiological and metabolic ramifications of heat-related illnesses. The pig is recognized as an excellent biomedical model (Prather et al. 2013) and the highly conserved metabolic and physiological responses to a severe heat load amongst species (Baumgard and Rhoads 2013) make it an ideal model to further our appreciation of thermal biology.
Typically, during inadequate nutrient intake AT is mobilized and NEFA contribution to whole-body oxidation is markedly increased (Vernon 1992). However, in this study, despite a 40% reduction in FI, HS pigs tended to have reduced basal NEFA concentrations and this suggests that they did not mobilize as much AT as PFTN counterparts. Furthermore, the NEFA response to the EC was blunted in the HS pigs and this reduced sensitivity to lipolytic signals agrees with our ruminant data (Baumgard et al. 2011). The decrease in circulating markers of AT mobilization during HS is mechanistically supported by a tendency for a reduction in ATGL gene expression, a key enzyme of the lipolytic cascade (Zimmermann et al. 2004), as well as the significant reduction in the gene encoding the AMPK regulatory subunit (PRKAG1). Interestingly, recent work has implicated AMPK in the regulation of ATGL content and lipase activity (Gaidhu et al. 2012), and it is tempting to speculate that their simultaneous reduction in transcript abundance is mechanistically related. The reduced capacity for AT to contribute to systemic energetics despite an increase in energy requirements during HS is consistent with research in agricultural animals and biomedical models (Torlinska et al. 1987; Shwartz et al. 2009; Pearce et al. 2013a). Moreover, similar to our ruminant data (Baumgard et al. 2011), the glucose response to the EC, an indicator of hepatic glycogenolysis capacity, was similar between HS and PFTN pigs. These data indicate that AT becomes refractory to adrenergic signals, whereas the liver remains responsive during HS. The mechanism for the different responsiveness between the liver and AT is unknown, but a rationale for it could be the increased reliance on glucose as a whole-body fuel during HS like we and others have demonstrated [see review (Baumgard and Rhoads 2013)]. By remaining sensitive to catabolic signals, the liver can maintain its pivotal role as the glucose supplier to extrahepatic tissues. Acute HS (24 h) increased plasma BHB, which is somewhat perplexing as circulating BHB is normally positively correlated with NEFA levels (Masoro 1977). However, this is not unprecedented as Ronchi et al. (Ronchi et al. 1999) reported a similar discordant NEFA and BHB pattern in HS ruminants. The fact that circulating BHB increases without a concomitant increase in circulating NEFA might indicate that ketone body oxidation is decreased; thereby reduce their plasma clearance. Interestingly, HS increases circulating LPS (presumably from intestinal origin as described later in this discussion) and we have recently demonstrated that LPS exposure reduces skeletal myoblast ketone oxidation (R. P. Rhoads and L. H. Baumgard, unpublished observation). Nonetheless, the mechanism by which HS uncouples the relationship between circulating NEFA and BHB and its energetic consequences require further investigation.
Reasons why heat-stressed animals fail to mobilize AT despite being in a hypercatabolic condition might be related to changes in insulin homeostasis. Insulin is a potent antilipolytic signal and is frequently elevated in HS animals when compared with PFTN counterparts (Baumgard and Rhoads 2013). In contrast, we did not observe treatment differences in basal insulin; however, C-peptide, a coproduct of insulin’s cleavage from proinsulin, was expression in LD and circulating LBP, both key proteins in the recognition of LPS by Toll-like receptor 4 (Lu et al. 2008), were upregulated and numerically increased, respectively, in HS pigs. Furthermore, the partial loss of differences in metabolism between treatments by d 6 of environmental exposure might correspond to the temporal pattern of acclimation by the intestinal barrier. This hypothesis is supported by the lack of differences in LBP by the end of the experiment. Moreover, because we utilized jugular catheters we administered antibiotics throughout the length of the experiment to prevent infection. This experimental approach may have influenced the intestinal flora, mitigating the leakage of luminal proinflammatory molecules to the portal and systemic blood stream, and/or energetic and nutrient demand of heat-stressed animals would presumably provide clues on how to better treat humans and animals suffering from heat-related illnesses.
Table 3. Effects of 8 days of pair-feeding or heat stress on gene expression in adipose tissue.
Table 4. Effects of 8 days of pair-feeding or heat stress on gene expression in skeletal muscle (Longissimus dorsi).
Table 5. Effects of 8 days of pair-feeding or heat stress on gene expression in liver.
In summary, HS represents a major environmental hazard compromising both human health and animal agriculture. In this study, we demonstrated that heat-stressed pigs experience increased basal insulin secretion and whole-body insulin sensitivity, and both variables likely prevent adipose tissue mobilization. The similarities with our observations during both growth and lactation in monogastrics and ruminants, coupled with the thermal therapy and diabetic models suggest that these responses are conserved amongst species, highlighting its importance in the adaptation to a thermal load. A better understanding of the physiological effects of HS is essential in order to develop treatment protocols and mitigation strategies both for human health and animal agriculture.
Acknowledgments
The authors express their appreciation to Anna Gabler, Samantha Lei, Sarah Edwards, Chris Guetzlaff, Heidi Reynolds, and Wes Schweer for their assistance in the live and laboratory phases of the experiment.
Conflict of Interest
The authors are not aware of any affiliation, memberships, funding, or financial holdings that might be perceived as affecting the objectivity of this review.
This article was originally published in Physiological Reports, 3 (2), 2015, e12315, doi: 10.14814/phy2.12315. This is an Open Access article under the terms of the Creative Commons Attribution License. References
Alamer, M. 2011. The role of prolactin in thermoregulation and water balance during heat stress in domestic ruminants. Asian J. Anim. Vet. Adv. 6:1153–1169.
Baumgard, L. H., and R. P. Rhoads. 2013. Effects of heat stress on postabsorptive metabolism and energetics. Annu. Rev. Anim. Biosci. 1:311–337.
Baumgard, L. H., J. B. Wheelock, S. R. Sanders, C. E. Moore, H. B. Green, M. R. Waldron, et al. 2011. Postabsorptive carbohydrate adaptations to heat stress and monensin supplementation in lactating Holstein cows. J. Dairy Sci. 94:5620–5633.
Bouchama, A., and J. P. Knochel. 2002. Heat stroke. N. Engl. J. Med. 346:1978–1988.
Brelje, T. C., and R. L. Sorenson. 1991. Role of prolactin versus growth hormone on islet B-cell proliferation in vitro: implications for pregnancy. Endocrinology 128:45–57.
Brockman, R. P., and B. Laarveld. 1986. Hormonal regulation of metabolism in ruminants; a review. Livest. Prod. Sci. 14:313–334.
Burdick Sanchez, N. C., R. Chaffin, J. A. Carroll, C. C. Jr Chase, S. W. Coleman, and D. E. Spiers. 2013. Heat-tolerant versus heat-sensitive Bos taurus cattle: influence of air temperature and breed on the metabolic response to a provocative immune challenge. Domest. Anim. Endocrinol. 45:180–186.
Changnon, S. A., K. E. Kunkel, and B. C. Reinke. 1996. Impacts and responses to the 1995 heat wave: a call to action. B. Am. Meteorol. Soc. 77:1497–1506.
Chung, J., A. K. Nguyen, D. C. Henstridge, A. G. Holmes, M. H. Chan, J. L. Mesa, et al. 2008. HSP72 protects against obesity-induced insulin resistance. Proc. Natl. Acad. Sci. USA 105:1739–1744.
Federation of Animal Sciences Societies. 2010. Guide for the care and use of agricultural animals in research and teaching. 3rd edition. www.fass.org, 2010.
Fuquay, J. W. 1981. Heat stress as it affects animal production. J. Anim. Sci. 52:164–174.
Furuyama, T., K. Kitayama, H. Yamashita, and N. Mori. 2003. Forkhead transcription factor FOXO1 (FKHR)-dependent induction of PDK4 gene expression in skeletal muscle during energy deprivation. Biochem. J. 375:365–371.
Gaidhu, M. P., G. Bikopoulos, and R. B. Ceddia. 2012. Chronic AICAR-induced AMP-kinase activation regulates adipocyte lipolysis in a time-dependent and fat depotspecific manner in rats. Am. J. Physiol. Cell Physiol. 303: C1192–C1197.
Gupte, A. A., G. L. Bomhoff, R. H. Swerdlow, and P. C. Geiger. 2009. Heat treatment improves glucose tolerance and prevents skeletal muscle insulin resistance in rats fed a high-fat diet. Diabetes 58:567–578.
Hall, D. M., K. R. Baumgardner, T. D. Oberley, and C. V. Gisolfi. 1999. Splanchnic tissues undergo hypoxic stress during whole body hyperthermia. Am. J. Physiol. 276: G1195–G1203.
Harris, R. A., M. M. Bowker-Kinley, B. Huang, and P. Wu. 2002. Regulation of the activity of the pyruvate dehydrogenase complex. Adv. Enzyme Regul. 42:249–259.
Hooper, P. L. 1999. Hot-tub therapy for type 2 diabetes mellitus. N. Engl. J. Med. 341:924–925.
Hughes, E., and C. Huang. 2011. Participation of Akt, menin, and p21 in pregnancy-induced beta-cell proliferation. Endocrinology 152:847–855.
Iguchi, M., A. E. Littmann, S. H. Chang, L. A. Wester, J. S. Knipper, and R. K. Shields. 2012. Heat stress and cardiovascular, hormonal, and heat shock proteins in humans. J. Athl. Train. 47:184–190.
Kahl, S., T. H. Elsasser, and J. W. Blum. 2000. Effect of endotoxin challenge on hepatic 5’-deiodinase activity in cattle. Domest. Anim. Endocrinol. 18:133–143.
Kahl, S., T. H. Elsasser, J. L. Sartin, and R. Fayer. 2002. Effect of progressive cachectic parasitism and growth hormone treatment on hepatic 5’-deiodinase activity in calves. Domest. Anim. Endocrinol. 22:211–221.
Kim, J. W., and C. V. Dang. 2006. Cancer’s molecular sweet tooth and the Warburg effect. Cancer Res. 66:8927–8930.
Kokura, S., S. Adachi, E. Manabe, K. Mizushima, T. Hattori, T. Okuda, et al. 2007. Whole body hyperthermia improves obesity-induced insulin resistance in diabetic mice. Int. J. Hyperthermia 23:259–265.
Kondo, T., K. Sasaki, R. Matsuyama, S. Morino-Koga, H. Adachi, M. A. Suico, et al. 2012. Hyperthermia with mild electrical stimulation protects pancreatic beta-cells from cell stresses and apoptosis. Diabetes 61:838–847.
Lambert, G. P., C. V. Gisolfi, D. J. Berg, P. L. Moseley, L. W. Oberley, and K. C. Kregel. 2002. Selected contribution: hyperthermia-induced intestinal permeability and the role of oxidative and nitrosative stress. J. Appl. Physiol. 92:1750– 1761; discussion 1749.
Larson, R. L. 2005. Effect of cattle disease on carcass traits. J. Anim. Sci. 83:E37–E43.
Leon, L. R., and B. G. Helwig. 2010. Heat stroke: role of the systemic inflammatory response. J. Appl. Physiol. (1985) 109:1980–1988.
Lu, Y. C., W. C. Yeh, and P. S. Ohashi. 2008. LPS/TLR4 signal transduction pathway. Cytokine 42:145–151. Masoro, E. J. 1977. Lipids and lipid metabolism. Annu. Rev. Physiol. 39:301–321.
Mullur, R., Y. Y. Liu, and G. A. Brent. 2014. Thyroid hormone regulation of metabolism. Physiol. Rev. 94:355–382.
National Research Council. 2012. Nutrient requirements of swine, 11th Revised ed. The National Academies Press, Washington, DC.
Niu, C. S., M. T. Lin, I. M. Liu, and J. T. Cheng. 2003. Role of striatal glutamate in heatstroke-induced damage in streptozotocin-induced diabetic rats. Neurosci. Lett. 348:77–80.
O’Brien, M. D., R. P. Rhoads, S. R. Sanders, G. C. Duff, and L. H. Baumgard. 2010. Metabolic adaptations to heat stress in growing cattle. Domest. Anim. Endocrinol. 38:86–94.
Pearce, S. C., N. K. Gabler, J. W. Ross, J. Escobar, J. F. Patience, R. P. Rhoads, et al. 2013a. The effects of heat stress and plane of nutrition on metabolism in growing pigs. J. Anim. Sci. 91:2108–2118.
Pearce, S. C., V. Mani, T. E. Weber, R. P. Rhoads, J. F. Patience, L. H. Baumgard, et al. 2013b. Heat stress and reduced plane of nutrition decreases intestinal integrity and function in pigs. J. Anim. Sci. 91:5183–5193.
Prather, R. S., M. Lorson, J. W. Ross, J. J. Whyte, and E. Walters. 2013. Genetically engineered pig models for human diseases. Annu. Rev. Anim. Biosci. 1:203–219.
Pucci, E., L. Chiovato, and A. Pinchera. 2000. Thyroid and lipid metabolism. Int. J. Obes. Relat. Metab. Disord. 24 (Suppl 2):S109–S112.
Ronchi, B., U. Bernabucci, N. Lacetera, A. Verini Supplizi, and A. Nardone. 1999. Distinct and common effects of heat stress and restricted feeding on metabolic status in Holstein heifers. Zootec. Nutr. Anim. 25:71–80.
Sanders, S. R., L. C. Cole, K. L. Flann, L. H. Baumgard, and R. P. Rhoads. 2009. Effects of acute heat stress on skeletal muscle gene expression associated with energy metabolism in rats. FASEB J. 23:598.597 (Abstr.).
Sanz Fernandez, M. V., S. C. Pearce, N. K. Gabler, J. F. Patience, M. E. Wilson, M. T. Socha, et al. 2014. Effects of supplemental zinc amino acid complex on gut integrity in heat-stressed growing pigs. Animal 8:43–50.
Sanz-Fernandez, M. V., S. C. Pearce, N. C. Upah, L. R. Long, A. Nayeri, E. Sucu, et al. 2012. Prolactin’s role during acute and chronic heat stress in growing pigs. FASEB J. 26:1079.1077 (Abstr.).
Seibert, J., R. Boddicker, J. Koltes, J. Reecy, D. Nettleton, M. Lucy, et al. 2014. Alterations in Transcriptional Profile As the Result of Prenatal Heat Stress Exposure in Pigs. Plant & Animal Genome XXII, San Diego, CA, P. P835.
Semenza, J. C., J. E. McCullough, W. D. Flanders, M. A. McGeehin, and J. R. Lumpkin. 1999. Excess hospital admissions during the July 1995 heat wave in Chicago. Am. J. Prev. Med. 16:269–277.
Shwartz, G., M. L. Rhoads, M. J. VanBaale, R. P. Rhoads, and L. H. Baumgard. 2009. Effects of a supplemental yeast culture on heat-stressed lactating Holstein cows. J. Dairy Sci. 92:935–942.
Stowell, R., T. Mader, and J. Gaughan. 2009. Environmental Management. in Livestock energetics and thermal environmental management. St Joshep, MI: Am Soc Agric Biol Eng, pp. 181–209.
St-Pierre, N. R., B. Cobanov, and G. Schnitkey. 2003. Economic losses from heat stress by US livestock industries. J. Dairy Sci. 86:E52–E77.
Torlinska, T., R. Banach, J. Paluszak, and A. Gryczka-Dziadecka. 1987. Hyperthermia effect on lipolytic processes in rat blood and adipose tissue. Acta. Physiol. Pol. 38:361–366.
Vernon, R. G. 1992. Effects of diet on lipolysis and its regulation. P. Nutr. Soc. 51:397–408.
Vives-Pi, M., N. Somoza, J. Fernandez-Alvarez, F. Vargas, P. Caro, A. Alba, et al. 2003. Evidence of expression of endotoxin receptors CD14, toll-like receptors TLR4 and TLR2 and associated molecule MD-2 and of sensitivity to endotoxin (LPS) in islet beta cells. Clin. Exp. Immunol. 133:208–218.
Wallace, T. M., J. C. Levy, and D. R. Matthews. 2004. Use and abuse of HOMA modeling. Diabetes Care 27:1487–1495.
Wheelock, J. B., R. P. Rhoads, M. J. Vanbaale, S. R. Sanders, and L. H. Baumgard. 2010. Effects of heat stress on energetic metabolism in lactating Holstein cows. J. Dairy Sci. 93:644–655.
Won, S., G. Xie, R. Boddicker, J. Rhoades, T. Scheffler, J. Scheffler, et al. 2012. Acute duration heat stress alters expression of cellular bioenergetic-associated genes in skeletal muscle of growing pigs. J. Anim. Sci. 95: 573 (Abstr.).
Zimmermann, R., J. G. Strauss, G. Haemmerle, G. Schoiswohl, R. Birner-Gruenberger, M. Riederer, et al. 2004. Fat mobilization in adipose tissue is promoted by adipose triglyceride lipase. Science 306:1383–1386.