Importance of studying transmission
Infectious diseases continue to have considerable impact on the health and well-being of individual animals and populations and on profitability of individual farms. Most commonly, frequency is measured by evaluating prevalence of infectious agents or evidence of exposure to them. Under certain conditions, incidence could also be calculated. However, for infectious diseases, it is also useful to understand how transmissible they are in a given population. One of the most widely used measures of transmission is the basic reproductive number (R0), defined as the average number of secondary infections resulting from a typical infectious individual in a completely susceptible population. Different versions of the reproductive number exist, depending on the assumptions used in its calculation and the phase of the epidemic. This measure continues to provide useful insights into different aspects of the epidemiology of infectious diseases and their control. One fundamental insight is the relationship between the R0 and the herd immunity threshold (Figure 1). The herd immunity threshold sets the target for the proportion of individuals that should be immunized in order to decrease incidence of infection. This has direct implications for long-term control of infections through vaccination, since many of our control strategies are based on regular vaccination of all or a subset of animals. In more general terms, this theoretical relationship provides an insight into the amount of effort that needs to be placed in the control of an infectious disease by applying different control measures 1. Reproductive numbers could also be used directly in dynamic models of disease transmission within populations to gain better understanding of disease circulation and appreciation of infection control strategies, or to monitor whether control measures are yielding results. Considerable progress has been made in public health and epidemiology of infectious diseases in people since the emergence of SARS, both in terms of extraction of parameters indicative of transmissibility and in the way experts are using them to design effective and sometimes most-efficient infection control strategies. Despite their usefulness for different purposes, their complementary nature to the measures of disease frequency, and sometimes abundance of data, such measures of transmission within swine populations are only beginning to emerge. Influenza in swine deserves to be studied in such a way because of its propensity to spread within and between pig populations and between different species. Influenza virus is often considered to be a virus with a relatively simple natural history, yet it continues to circulate endemically in many swine populations with oscillations that are poorly understood. It is well established that the density of pigs in the area is associated with the likelihood of infection in herds, yet little is known about the exact conditions that facilitate such transmission. It is also considered as a zoonotic infection, but a clearer definition of what type of zoonosis influenza is would be useful for risk assessment and communication purposes. This contribution will therefore focus on a brief review of some interesting recent developments for influenza in swine and other species as it has impact on requirements for data collection and analysis.
Figure 1. Theoretical relationship between herd immunity threshold and the basic reproductive number. This relationship indicates that infections with higher reproductive numbers require greater herd immunity for their longterm control.
Measures of influenza transmissibility within swine herds and sources of data
Measures of transmissibility are just starting to emerge in the scientific literature for influenza virus in swine populations. Early reports were based on theoretical reasoning, rather than observed data 2. More recently, estimates of reproductive numbers were based on real data obtained from experiments and observational studies. These numbers are summarized in Figure 1 3-5 using fixed effect meta-analysis. It is clear that estimates of reproductive numbers are quite variable. The sources of this variability should be studied further, and this could lead to further understanding of transmission. However, it should also be noted that heterogeneity in reproductive numbers among studies is expected and depends on the contact patterns in populations, inter alia. Heterogeneity in reproductive numbers has been observed both for SARS 6 and for influenza in people and is therefore not surprising. It should not prevent us from adapting such approaches in studying influenza and other infectious diseases in swine. It is interesting to note that all of the current estimates of R0 are obtained using detailed longitudinal measurements of individual animals under field or experimental conditions. While this will likely remain the most accurate method of studying transmissibility, it should be noted that reproductive numbers and other important epidemiological parameters in public health have frequently been obtained from less than perfect data, often just the case numbers in the early phases of the outbreak. For example, the first 205 probable cases of SARS were used to learn about the epidemiology of this disease in 20037. Similarly, case numbers obtained using active or passive surveillance during the 1918 influenza pandemic under different settings have been used to establish the magnitude of R08.
With detailed computerized records being present on many sow and growing pig sites, we should have data that could be used, under certain conditions, to inform us about transmissibility. Examples could include records of abortions in confirmed new incursions of influenza and possibly records of mortality over time due to respiratory signs in cases when influenza virus is confirmed and other pathogens are not involved. Caution must be exercised, since influenza virus in swine rarely cause significant mortality9.
Figure 2. Fixed effect meta-analysis of recent observational studies and transmission experiments reporting estimates of the basic reproductive number. Estimate of the overall R0 has been provided for illustration purposes, but the value of I2 suggests large heterogeneity among studies.
Transmission of influenza virus within herds
Role of duration of different periods
Understanding the transmission pattern of influenza in swine populations should be based on a thorough understanding of the natural history of influenza virus infection at the individual animal level. Unlike many other infections in swine populations, influenza has a relatively simple natural history. Under experimental conditions, the period between infection and becoming infectious is 24 hours 9, and the period that a pig sheds the virus in considerable amounts is 5-7 days9. Duration of effective immunity over a prolonged period is not well understood10. The majority of dynamic models that aim to study influenza in other species assume that influenza virus infection will produce long-term immunity and that immune individuals will remain immune 11. This gives rise to a susceptible-exposed-infectiousresistant (SEIR) class of dynamic models. This may be a reasonable assumption for studies that aim to evaluate control measures over a relatively short time period in these populations. Such an assumption is probably reasonable for our growing pigs, but perhaps needs to be considered carefully in sow herds. Figure 3 uses a very simple deterministic model, based on simplifying assumptions to illustrate the point that assumption about duration of immunity in sow populations, coupled with an inherently large replacement rate, could have large implications on our understanding of influenza virus circulation. In the case that influenza is assumed to produce a life-long immunity, a single introduction of a virus will result in an outbreak, which will be followed by the fade-out of the virus from this population. In contrast, if we assume that the average duration of immunity is 1 year, we can expect repeated waves of virus circulation due to infection from the sow population alone, without consideration for the role of circulation in suckling piglets. This perhaps illustrates one additional point: a new clinical outbreak due to infectious disease in a sow herd is frequently ascribed to external breaches in biosecurity. However, at least some of these outbreaks could be due to the natural cycle of infection in an inherently open population and the role that duration of immunity plays.
Figure 3. Expected circulation of influenza virus in a sow herd under the assumptions of permanent immunity and waning immunity. Assumptions: (i) sow herd size of 2000 sows, (ii) homogeneous mixing, (iii) R0=3, (iv) annual replacement rate of 40%, (v) all replacement animals are susceptible, (vi) no contribution of other production classes including suckling piglets (vii) preinfectious period is 1 day, (viii) average infectious period is 6 days. Legend: (i) Infect: perm = number of infectious sows at a point in time under the assumption of permanent immunity, (ii) Infectwan = number of infectious sows at a point in time under the assumption of waning immunity, (iii) Suscept: perm = number of susceptible sows at a point in time under the assumption of permanent immunity, (iv) Suscept: wan = number of susceptible sows at a point in time under the assumption of waning immunity.
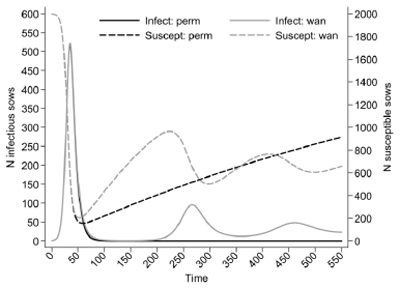
Many influenza control strategies are based on maximizing maternal immunity, which might be present in growing pigs until the late nursery stage. Marked reduction in transmission was reported when piglets were exposed to a virus identical to the influenza virus used to immunize sows4. This finding concurs well with the rationale of practitioners who employ this strategy to control influenza virus infection in some herds. In the same study, transmission was slightly reduced when piglets with maternally derived antibodies were exposed to a heterologous virus of the same subtype. At the nucleotide sequence level, the similarity of the hemagglutinin of H1N1 between the virus used for immunization and for challenge was 86%. However, the role of maternal immunity for transmission is also subject to some uncertainty. Authors of a recent observational cohort study reported a high circulation of influenza viruses around weaning time in one of the study farms12. In the latter study, piglets had influenza virus positive nasal swabs, despite the presence of a high level of antibodies in their sera. It was therefore postulated that infection could occur in the presence of the colostrum-derived immunity. In another observational study, pigs infected in the presence of maternal antibodies did not seroconvert 5, a finding in agreement with previous experimental work13. This could have implications on the circulation of influenza virus in populations of growing pigs, since maternal immunity may persist on average approximately 8 weeks14, with large variability among individual animals. This time represents a substantial proportion of the productive life of a growing pig. Figure 4 contrasts the expected circulation of influenza virus under two different scenarios in a batch of growing pigs farrowed in a farrowing cohort with 80% of sows immune. The first scenario is based on the assumption of complete protection of maternal immunity against infection in piglets that received maternal antibodies. The second scenario is based on the assumption that piglets will be completely susceptible to infection in the presence of maternal antibodies, and will not develop active immunity after they stop shedding the virus. Although both of these scenarios are unrealistic, they were made deliberately simplistic with the intention to show how maternal immunity could shape the circulation of influenza virus in a closed population. In the former scenario, only the initial proportion of susceptible animals allowed the virus to cause infection in the later phase of the growing period, otherwise circulation would have been stopped completely; whereas in the latter scenario the circulation of the virus starts early with a higher peak. More studies under field conditions would be useful to better understand the circulation of influenza viruses in this early phase of the pig’s life.
Figure 4. Expected circulation of influenza virus based on a deterministic model in a closed cohort of a growing pig herd under the assumption that maternally derived antibodies offer complete protection, or that maternally derived antibodies (MDAs) do not offer any resistance against infection and do not allow development of active immunity. Assumptions: (i) 1000 pigs in a batch, (ii) homogeneous mixing, (iii) R0=3, (iv) no contribution of other production classes or batches (v) preinfectious period is 1 day, (vi) average infectious period is 6 days (vii) only 80% of the pigs at time 0 are maternally immune (20% of piglets are farrowed in parity 1 litters and do not have any antibodies), (viii) one infectious pig introduced at time 0. Legend: (i) Infect: immunity = number of infectious growing pigs at a point in time under the assumption that MDAs offer full protection, (ii) Infect: no immunity = number of infectious growing pigs at a point in time under the assumption that MDAs do not offer protection against infection and do not allow development of active immunity, (iii) Suscept: immunity = number of infectious growing pigs at a point in time under the assumption that MDAs offer full protection, (iv) Suscept: no immunity = number of infectious growing pigs at a point in time under assumption that MDAs do not offer protection against infection and do not allow development of active immunity.
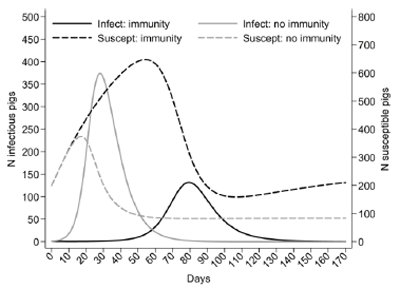
Often times, several waves of influenza virus circulation have been reported, both in the scientific literature 5,12,15 and circumstantially, based on clinical impression. The easiest explanation would be to assume that different animals are becoming infected at different times. Although this might be a plausible explanation in some situations, it is puzzling that a recent study identified pigs that with repeated infection with influenza virus after an interval of >4 weeks. The viruses were very similar, and in some cases identical when partial nucleotide sequences of the HA gene were compared.
Role of concurrent circulation of different influenza viruses and different co-infections
Exposure of pigs to different subtypes and variants occurs frequently 16. In addition, co-circulation of viruses has been documented in the same batch of pigs at different times or simultaneously5,12. This has obvious implications on the pattern of influenza circulation in a barn. Co-infections have been documented in the same animals as well, which additionally is a necessary condition for reassortment to occur. In fact, a novel recombinant was detected in a study that involved relatively few herds5. This is an interesting addition to a recent study which reported emergence of one recombinant virus per 2-3 years for Eurasian variants measured between 1982 and 2008; before the emergence of pandemic H1N117. In North American herds, multiple recombinants were reported shortly after introduction of pH1N118 and their co-circulation in specific well-defined populations should be studied further. Concurrent infections with influenza virus and other respiratory pathogens are also frequent under field conditions. Involvement of influenza virus in porcine respiratory disease complex has been well described. Less is known about the influence that concurrent infections could have on epidemiological parameters of importance for dynamics of influenza at the population level. An interesting perspective comes from a recent comparison between nonimmunocompromised and severely immunocompromised people who were sampled daily for influenza virus until cessation of shedding. The mean duration of shedding in the severely immunocompromised patients was 19 days, as compared to ~6.4 days in the nonimmunocompromised19.
Role of contacts between different age groups
In a longitudinal study of influenza virus circulation in finisher pigs, Loeffen et al reported different patterns of influenza virus circulation between finishing pigs raised in farrow-to-finish herds and in specialized finishing sites20. Incidence of influenza was highest at the beginning of the finishing period in farrow-to-finish sites, and at the end of the finishing period for pigs in specialized finishing operations. This high incidence at the beginning of the finisher period was ascribed to a higher degree of contact between finisher pigs and sows or weaned pigs in operations that house all production classes on the same site and the resulting transmission between them. This finding explains why many farrow-to-finish sites have ongoing circulation of influenza virus. It remains a bit puzzling why some large production systems with much better age separation also have endemic circulation of influenza viruses. At a very general level, the answer is probably in the interaction between: (i) large sow herds where pockets of susceptible animals might allow long-term maintenance of the virus, (ii) mixing of animals from different sow herds with varying degrees of immunity, which might allow maintenance of the virus in a population over longer period of time, and (iii) degree of contact between different age groups during the nursery and finisher phase. It is not uncommon to have more than one nursery or finisher barn on specialized nursery or finisher sites. The reality of some production systems is that there is some overlap between different age groups in different buildings, and this could be a factor contributing to endemic circulation of influenza virus. In order to design more effective infection control strategies for influenza virus circulation, we need to have a better understanding of transmission not only within populations, but also between different production classes. Recent publications also point in this direction, particularly transmission in a sow herd5,12.
Role of environment and seasonality of influenza
Seasonality of any infectious disease has implications for its transmission and is an important factor when designing control programs. In temperate regions, seasonal influenza in people peaks in the winter months. Contributors to seasonality are still not completely understood although several observational and experimental studies pointed to the role of humidity and temperature in the seasonality21-24. Lowen et al 22 in a guinea pig model showed that the relationship between transmission probability due to aerosol and relative humidity was not linear. The highest transmission occurred during periods of low relative humidity, decreasing at moderate humidity, then increasing again at 65%, and ceasing at a relative humidity of 80%. The authors concluded that aerosol transmission is most efficient in the experimental model of guinea pigs in conditions of low humidity and low environmental temperature. Interestingly, low temperature also extended the period of viral shedding. Contrary to this, high humidity did not have an impact on the contact spread between experimental animals25, which led these authors to propose that aerosol transmission plays an important role among humans in temperate regions, whereas contact spread is more important in tropical zones. In contrast, the existence of influenza seasonality in swine populations is not well understood. The general consensus is that a seasonal pattern diminished with the advent of modern swine production systems10. One study that explicitly addressed influenza seasonality in pigs was conducted recently in four European countries between 2006 and 2008. No evidence of between-season differences in proportion of influenza-exposure positive pigs or farms could be found on the basis of sampling that was restricted to summer and winter periods16.
Swine barns are located in different climate regions, and modern production systems have sophisticated systems of environmental control. Microclimate conditions such as temperature and relative (and absolute) humidity could have important implications for spread within different barns and production classes and deserves to be studied more thoroughly.
Transmission between populations located on different sites
Animal density in an area and related indicators have often been considered as a risk factor for prevalent cases of influenza, but little is known about the actual mechanisms of transmission. Some insight may be gained from the recent Australian outbreak of equine influenza, both in terms how the data were recorded and analyzed and conclusions reached. Of importance in this outbreak was the tracing of contacts and accurate assessment of the times when clinical outbreaks occurred. Use of social network analysis and proximity analysis provided some insight into the nature of spread in the early phases of the epidemic 26, whereas time-varying covariates assessed within survival analysis models provided an insightful perspective of the role of environmental factors in the disease spread once movement restriction was in control27. Interestingly, the latter study identified the role of time lagged relative humidity and temperature for incident cases of influenza in horse holdings, which was in line with epidemiological and experimental findings of the association between influenza circulation and humidity in human populations. Although it is unrealistic to expect that cases of influenza infections in swine herds will be investigated with the same thoroughness as the cases of a reportable disease, equine influenza, in a completely susceptible population, these reports contain some important lessons for data acquisition and analysis. It is encouraging to see a wide acceptance of the use of premises identification in different North American jurisdictions, either for the purposes of tracing outbreaks or disease control programs. Ultimately it could lead to development of real-time surveillance systems that are based on both spatial and network information. These types of epidemiological models for studying transmission will become a necessity in our highly integrated production systems.
Zoonotic transmission potential of Influenza A virus in pigs
Influenza A virus is a viral pathogen with high relevance for public health due to its zoonotic potential, reflected either in increased transmission events between animals and people, the virulence of some recently emerged variants in human populations, or both. Examples of emerging zoonotic influenza viruses are becoming more frequent in recent times. This includes, the highly pathogenic H5N1 (HP H5N1)28, and H7N9 influenza virus which emerged recently in China29. In North America, swine-origin variant H3N2 virus in the USA caused over 300 human cases in 2012, most of them in people with prolonged exposure to swine during pig shows30. Instances of person-to-person transmissions have been postulated in some outbreaks of H3N2 virus. The 2009 pandemic H1N1 virus was linked with swine on the basis of genetic information31, and between-species transmission has been reported in both directions 32-34. Prior to emergence of pH1N1, a number of swine-originating reassortant H1N1 viruses caused spillover infections in people35,36. Thus, it is of great interest to understand transmissibility of influenza viruses between animals and people, and then subsequently between people. Wolfe et al 37 proposed a useful method to classify zoonoses with respect to this potential. According to this classification, pathogens that transmit from animals to people and then continue to spread efficiently among people have the potential to cause major epidemics in people and are considered to be stage IV zoonoses. Based on the same classification, stage II zoonoses are diseases transmitted between animals and humans, but not between people; whereas a stage III zoonosis will lead to some secondary transmission between people, potentially leading to local outbreaks. This classification was further supplemented by considering the person-to-person transmissibility expressed in terms of the reproductive number in human populations38. From this perspective, stage II, III, and IV zoonoses have R=0, <1, and ≥ 1, respectively.
The transmissibility of pathogens in different populations could be assessed using a variety of approaches, including virological studies, transmission experiments, and field studies based on seroprevalence studies and on the basis of data observed during field investigations. Methods for evaluation of self-limited (ie, stuttered) transmission chains, typical for stage III zoonoses, are still under development, but have received heightened interest in recent times; a recent overview summarized developments in this area39. De Serres et al40 initially proposed three approaches to study the transmissibility of communicable diseases close to elimination, a situation that is similar to emergence of stage III zoonoses, via the: (i) proportion of imported cases, (ii) distribution of size of outbreaks, and (iii) distribution of duration of outbreaks. The approaches used were based on an assumed infinite pool of susceptible individuals and are, therefore, particularly limited in well-defined closed populations. Ferguson et al41 proposed a method based on the number of animal-to-human cases observed, the number of clusters recorded, and the size of the largest outbreak. Further modifications to this methodology were proposed42. Using the proposed methodology, it was estimated that the most likely estimate for R0 for H3N2 swine-origin viruses without the M segment from the 2009 pH1N1 was 0.2, and for H3N2 swine-origin viruses with the M segment was 0.542. This can be used directly in risk assessment. Figure 5 shows the expected size of outbreaks (ie, number of people involved in a single transmission chain) under the assumption that R=0.5. It can be seen that most transmissions will result in infection in a single individual; however there is also a small probability that such transmission could result in a chain that will lead to larger number of individuals before it dies out.
Figure 5. Expected outbreak size in a human population under the assumption that the reproductive number is 0.5, based on 1000 simulations.
Conclusions
Understanding transmission of influenza within and between populations serves to directly inform the infection control strategies at different levels. Recent longitudinal field studies provided interesting insight into circulation of influenza virus under field conditions. Similar studies are needed under different conditions in populations of growing pigs, and particularly in sows. For such investigations, tools of molecular and infectious disease epidemiology should be used and further developed. In that respect, estimation of parameters that measure transmissibility would be particularly welcomed. For transmission between populations, advantage should be taken of analytical approaches which consider spatial and network characteristics of the populations. For this to occur, however, improved quality of field data is needed. Use of unique identifiers to link data among different data sources will continue to be a necessary requirement and the first step towards that.
References
1. Goldstein E, Paur K, Fraser C, Kenah E, Wallinga J, Lipsitch M. Reproductive numbers, epidemic spread and control in a community of households. Math Biosci 2009; 221: 11-25.
2. Saenz RA, Hethcote HW, Gray GC. Confined animal feeding operations as amplifiers of influenza. Vector Borne Zoonotic Dis 2006; 6: 338-46.
3. Romagosa A, Allerson M, Gramer M, et al. Vaccination of influenza a virus decreases transmission rates in pigs. Vet Res 2011; 42: 120,9716-42-120.
4. Allerson M, Deen J, Detmer SE, et al. The impact of maternally derived immunity on influenza A virus transmission in neonatal pig populations. Vaccine 2013; 31: 500-5.
5. Rose N, Herve S, Eveno E, et al. Dynamics of influenza A virus infections in permanently infected pig farms: evidence of recurrent infections, circulation of several swine influenza viruses and reassortment events. Vet Res 2013; 44: 72,9716-44-72.
6. Bauch CT, Lloyd-Smith JO, Coffee MP, Galvani AP. Dynamically modeling SARS and other newly emerging respiratory illnesses: past, present, and future. Epidemiology 2005; 16: 791-801.
7. Lipsitch M, Cohen T, Cooper B, et al. Transmission dynamics and control of severe acute respiratory syndrome. Science 2003; 300: 1966-70.
8. Vynnycky E, Trindall A, Mangtani P. Estimates of the reproduction numbers of Spanish influenza using morbidity data. Int J Epidemiol 2007; 36: 881-9.
9. Janke BH. Influenza A Virus Infections in Swine: Pathogenesis and Diagnosis. Vet Pathol 2013;.
10. Van Reeth K, Brown IH, Olsen CW. Influenza virus. In: Zimmerman J, Karriker L, Ramirez A, Schwartz K, Stevenson G, editors. Diseases of swine.: Wiley-Blackwell; 2012. p. 557-571.
11. Tuite AR, Fisman DN, Kwong JC, Greer AL. Optimal pandemic influenza vaccine allocation strategies for the Canadian population. PLoS One 2010; 5: e10520.
12. Simon-Grife M, Martin-Valls GE, Vilar MJ, et al. Swine influenza virus infection dynamics in two pig farms; results of a longitudinal assessment. Vet Res 2012; 43: 24,9716-43-24.
13. Loeffen WL, Heinen PP, Bianchi AT, Hunneman WA, Verheijden JH. Effect of maternally derived antibodies on the clinical signs and immune response in pigs after primary and secondary infection with an influenza H1N1 virus. Vet Immunol Immunopathol 2003; 92: 23-35.
14. Loeffen WL, Nodelijk G, Heinen PP, van Leengoed LA, Hunneman WA, Verheijden JH. Estimating the incidence of influenza-virus infections in Dutch weaned piglets using blood samples from a cross-sectional study. Vet Microbiol 2003; 91: 295-308.
15. Allerson MW, Davies PR, Gramer MR, Torremorell M. Infection Dynamics of Pandemic 2009 H1N1 Influenza Virus in a Two-Site Swine Herd. Transbound Emerg Dis 2013.
16. Kyriakis CS, Rose N, Foni E, et al. Influenza a virus infection dynamics in swine farms in Belgium, France, Italy and Spain, 2006-2008. Vet Microbiol 2013; 162: 543-50.
17. Lycett SJ, Baillie G, Coulter E, et al. Estimating reassortment rates in co-circulating Eurasian swine influenza viruses. J Gen Virol 2012; 93: 2326-36.
18. Ducatez MF, Hause B, Stigger-Rosser E, et al. Multiple reassortment between pandemic (H1N1) 2009 and endemic influenza viruses in pigs, United States. Emerg Infect Dis 2011; 17: 1624-9.
19. Memoli MJ, Athota R, Reed S, et al. The Natural History of Influenza Infection in the Severely Immunocompromised vs Nonimmunocompromised Hosts. Clin Infect Dis 2014; 58: 214-24.
20. Loeffen WL, Hunneman WA, Quak J, Verheijden JH, Stegeman JA. Population dynamics of swine influenza virus in farrow-to-finish and specialised finishing herds in the Netherlands. Vet Microbiol 2009; 137: 45-50.
21. Tamerius JD, Shaman J, Alonso WJ, et al. Environmental predictors of seasonal influenza epidemics across temperate and tropical climates. PLoS Pathog 2013; 9: e1003194.
22. Lowen AC, Mubareka S, Steel J, Palese P. Influenza virus transmission is dependent on relative humidity and temperature. PLoS Pathog 2007; 3: 1470-6.
23. Shaman J, Kohn M. Absolute humidity modulates influenza survival, transmission, and seasonality. Proc Natl Acad Sci U S A 2009; 106: 3243-8.
24. Shaman J, Pitzer V, Viboud C, Lipsitch M, Grenfell B. Absolute Humidity and the Seasonal Onset of Influenza in the Continental US. PLoS Curr 2009; 2: RRN1138.
25. Lowen AC, Steel J, Mubareka S, Palese P. High temperature (30 degrees C) blocks aerosol but not contact transmission of influenza virus. J Virol 2008; 82: 5650-2.
26. Firestone SM, Christley RM, Ward MP, Dhand NK. Adding the spatial dimension to the social network analysis of an epidemic: investigation of the 2007 outbreak of equine influenza in Australia. Prev Vet Med 2012; 106: 123-35.
27. Firestone SM, Cogger N, Ward MP, Toribio JA, Moloney BJ, Dhand NK. The influence of meteorology on the spread of influenza: survival analysis of an equine influenza (A/H3N8) outbreak. PLoS One 2012; 7: e35284.
28. Li KS, Guan Y, Wang J, et al. Genesis of a highly pathogenic and potentially pandemic H5N1 influenza virus in eastern Asia. Nature 2004; 430: 209-13.
29. Lam TT, Wang J, Shen Y, et al. The genesis and source of the H7N9 influenza viruses causing human infections in China. Nature 2013.
30. Centers for Disease Control and Prevention (CDC). Influenza A (H3N2) variant virus-related hospitalizations: Ohio, 2012. MMWR Morb Mortal Wkly Rep 2012; 61: 764-7.
31. Smith GJ, Vijaykrishna D, Bahl J, et al. Origins and evolutionary genomics of the 2009 swine-origin H1N1 influenza A epidemic. Nature 2009; 459: 1122-5.
32. Howden KJ, Brockhoff EJ, Caya FD, et al. An investigation into human pandemic influenza virus (H1N1) 2009 on an Alberta swine farm. Can Vet J 2009; 50: 1153-61.
33. Pasma T, Joseph T. Pandemic (H1N1) 2009 infection in swine herds, Manitoba, Canada. Emerg Infect Dis 2010; 16: 706-8.
34. Forgie SE, Keenliside J, Wilkinson C, et al. Swine outbreak of pandemic influenza A virus on a Canadian research farm supports human-to-swine transmission. Clin Infect Dis 2011; 52: 10-8.
35. Shinde V, Bridges CB, Uyeki TM, et al. Triple-reassortant swine influenza A (H1) in humans in the United States, 2005-2009. N Engl J Med 2009; 360: 2616-25.
36. Belser JA, Gustin KM, Maines TR, et al. Pathogenesis and transmission of triple-reassortant swine H1N1 influenza viruses isolated before the 2009 H1N1 pandemic. J Virol 2011; 85: 1563-72.
37. Woolhouse ME, Gowtage-Sequeria S. Host range and emerging and reemerging pathogens. Emerg Infect Dis 2005; 11: 1842-7.
38. Lloyd-Smith JO, George D, Pepin KM, et al. Epidemic dynamics at the human-animal interface. Science 2009; 326: 1362-7.
39. Blumberg S, Lloyd-Smith JO. Comparing methods for estimating R0 from the size distribution of subcritical transmission chains. PLoS Comput Biol 2013; 5: 131-45.
40. De Serres G, Gay NJ, Farrington CP. Epidemiology of transmissible diseases after elimination. Am J Epidemiol 2000; 151: 1039,48; discussion 1049-52.
41. Ferguson NM, Fraser C, Donnelly CA, Ghani AC, Anderson RM. Public health. Public health risk from the avian H5N1 influenza epidemic. Science 2004; 304: 968-9.
42. Cauchemez S, Epperson S, Biggerstaff M, Swerdlow D, Finelli L, Ferguson NM. Using routine surveillance data to estimate the epidemic potential of emerging zoonoses: application to the emergence of US swine origin influenza A H3N2v virus. PLoS Med 2013; 10: e1001399.