Introduction
Influenza A virus infections (flu) in swine cause respiratory disease and decreased growth performance (Olsen et al., 2006). Different studies have demonstrated that flu is widespread in swine throughout the world, and the constant risk of virus transmission, especially to humans (Maes et al., 2000; Jung et al., 2002; Hayden and Croisier, 2005; Maldonado et al., 2006; Myers et al., 2007; Poljak et al., 2008), calls for the need to have a better understanding of influenza transmission in pigs.
Influenza A virus can be transmitted through direct nose-to-nose contact and aerosols; however, information on flu transmission in pig populations is scarce (Brown, 2000). Neonatal pigs have been shown to play a key role in the transmission of influenza viruses within and between herds in that they represent a continual reservoir of infectious virus within breeding herds and a source for grow-finish sites (Allerson and Torremorell, 2010). Dam vaccination is a common approach for controlling influenza virus in swine breeding herds. In North America, pre-farrow dam vaccination is performed in 70% of the herds having 500 or more sows (NAMHS, 2007). Dam vaccination is directed at protecting breeding animals from reproductive losses such as abortions and returns to oestrus (Vannier, 1999; Karasin et al., 2000). More importantly, dam vaccination aims at conferring maternal- derived antibodies (MDA) to the newborn piglet (Thacker and Janke, 2008) to reduce clinical signs upon infection. Research suggests that pigs with MDA and challenged with homologous (Loeffen et al., 2003) and heterologous (Kitikoon et al., 2006) strains can be partially protected against influenza-like clinical signs although viral shedding might not appear to be influenced by the presence of MDA (Loeffen et al., 2003; Kitikoon et al., 2006). In contrast, other reports suggest that homologous maternal immunity achieved in neonatal pigs through dam vaccination and ingestion of colostrum can reduce the transmission of influenza virus in neonatal pig populations (Allerson et al., 2011). Overall, the degree that MDA impact the transmission of influenza viruses is not well understood and needs further evaluation.
Recent reports have also highlighted the importance of aerosol transmission, not only in humans (Tellier, 2009), but also in different species such as ferrets (Munster et al., 2009), guinea pigs (Lowen et al., 2006) and chickens (Yee et al., 2009). Airborne influenza transmission is considered important in pigs, although the data on swine influenza virus are scarce. There is anecdotal and epidemiological information that implicates pigs as the virus source for neighbouring turkey flocks (Choi et al., 2004; Corzo et al., 2011a). Furthermore, epidemiological studies (Maes et al., 2000; Poljak et al., 2008) have also concluded that pig farm density is a risk factor for influenza seropositive pig herd status pointing at the airborne route as the likely route for regional virus spread. Recently, swine influenza virus could be detected in the air from experimentally infected pigs (Loeffen et al., 2011). Characterization of infectious aerosols was further evaluated in acutely infected pigs showing a strong correlation between presence of influenza virus in the air and the number of shedding pigs in a population (Corzo et al., 2011b).
However, the impact of maternally derived immunity on influenza virus transmission, specifically aerosol transmission, in swine has not been assessed. Therefore, the objective of this study was to determine whether pigs with MDA are able to generate detectable levels of infectious influenza aerosols under experimental conditions.
Materials and Methods
All procedures were conducted in accordance with the University of Minnesota Institutional Biosafety Committee (IBC) and Institutional Animal Care and Use Committee (IACUC).
Study site
The study was performed at the University of Minnesota College of Veterinary Medicine animal research facilities (St. Paul, MN, USA). The facility is a BSL-2 research unit equipped with showers and a negative pressure ventilation system with dedicated materials and personnel. For the purpose of this study, enhanced personal biosecurity measures were followed, which included the use of room-specific personal protective equipment including N-95 respirators, goggles, gloves, hairnets and dedicated clothing and footwear.
Each isolation room had a utility area of 2.08 m2 where the feed bin and cleaning tools were stored and one housing pen of 7.28 m2. The utility area and the pen were separated by a metal gate. The walls were made of brick and tile and the floor was solid concrete. Each pen had one water line with two water nipples. The distance between the floor and the ceiling was 3.75 m, and the total airspace volume was 35.1 m3 per room.
Study animals
Thirty-three individually identified pigs from an influenza virus-free herd were used in this study. Prior to the start of the study, the herd had been serologically tested and deemed negative for porcine reproductive and respiratory syndrome virus (PRRSv), Mycoplasma hyopneumoniae and influenza A virus. Pigs were derived from sows that had been either vaccinated or un-vaccinated (controls). Eight sows were divided into three groups in which three sows were vaccinated 38 and 17 days pre-farrow with a killed triple reassortant influenza virus, A/Swine/IA/00239/2004 (H1N1) belonging to the H1 beta cluster (VAC-HOM); three different sows were vaccinated at the same time with a killed triple reassortant influenza virus A/Swine/IL/ 02450/2008 (H1N1) belonging to the H1 alpha cluster (VAC-HET). The viruses used in the vaccines for the VACHOM and VAC-HET groups were genetically distinct and shared 86% hemagglutinin (HA) nucleotide gene similarity. The remaining sows were left unvaccinated and served as the source for control (CTRL) and seeder pigs. Upon farrowing, piglets were ear-tagged and remained with their biological mother until weaning. Piglets were handled following standard farm production practices. Blood samples were collected from piglets and tested for influenza antibodies via hemagglutination inhibition (HI) (Pedersen, 2008) using the challenge virus (A/Swine/IA/00239/2004 H1N1) and enzyme linked immunosorbent assay (ELISA) (IDEXX Flock ChekTM AI MultiS-Screen; IDEXX Laboratories, Westbrook, ME, USA) (Ciacci-Zanella et al., 2010).
Each group consisted of 11 pigs total – 10 pigs born from sows vaccinated homologously, heterologously or unvaccinated plus one 'seeder pig' born from unvaccinated sows. Pigs within groups were selected based on HI titres. Pigs with the highest HI titres in each litter were included in the study. Upon weaning, pigs were transported to the isolation animal unit at the University of Minnesota and were housed in separate rooms based on their treatment allocation. Space allowance was 0.58 m2 per pig and pigs had ad-libitum access to feed and water throughout the study. Nasal swabs from all piglets were collected on the same day and tested for influenza RNA using real-time reverse transcriptase PCR (RRT-PCR) targeting the matrix gene (Spackman et al., 2002; Spackman and Suarez, 2008) as described below.
Air sample collector
Air was sampled using a liquid cyclonic collector (Midwest Micro Tek, Brookings, SD, USA) previously validated for the detection of airborne influenza A virus. Limit of detection was previously estimated at 10 TCID50/ml (Corzo et al., 2011b). Briefly, the sampling device is comprised of three components: (i) an electric power source, (ii) a turbine powered by an electric motor which is attached to an aluminium four-legged structure and (iii) a removable aluminium-plastic collection vessel in which liquid media is placed for sample collection (Fig. 1). When the collector is running, air is sucked in from underneath the collection vessel into the collection vessel containing liquid media. This turbulence creates the mixing effect between the air and the liquid media. Once the air hits the diagonal collection vessel wall, the air is forced to exit upwards through circular openings located on the aluminium four-legged structure. This effect results in particles present in the air being washed with liquid media. This device is able to process 400 l of air per minute. Three separate devices were used in our study and randomly assigned to a specific room.
Experimental inoculation and exposure
The seeder pig from each group was taken to a separate isolation room for challenge inoculation. Pigs were sedated by an intramuscular injection with a rapid-acting anaesthetic (Telazol; Fort Dodge Animal Health, Fort Dodge, IA, USA) at a dose of 6.6 mg/kg. Once pigs were sedated, they were manually restrained and the oral cavity exposed. A catheter attached to a syringe was used to deliver 0.5 ml of A/Swine/IA/00239/2004 (H1N1) influenza A virus containing 1x 107 TCID50/ml into the trachea. Additionally, 0.25 ml of the same inoculum was delivered into each of the nostrils. Nasal swabs of seeder pigs were collected at 24 and 48 h post-inoculation and tested for influenza virus RNA by RRT-PCR. Upon confirmation of infection, seeder pigs were returned to their original room and commingled with the rest of the study (contact) pigs. Pigs were observed daily, and individual nasal swabs were collected from all pigs in the room until the study ended at 10 days post-contact exposure. The seeder pig remained in the room with the rest of the pigs throughout the duration of the study.
Clinical signs
Influenza-like clinical signs consisting of cough, nasal discharge and sneezing were recorded daily throughout the study. Pigs were observed during 10 min by the same person, and presence or absence of clinical signs was noted.
Personnel flow and air sampling process
A total of 3 air samples were collected each day in each group for a total of 10 days. Samples were collected every eight hours at approximately 6:00 AM, 2:00 PM and 10:00 PM. Personnel movement between rooms required the use of room-specific coveralls, hairnets, gloves, N-95 masks and boots. Personnel would start the sampling process by first entering the room, housing the VAC-HOM, followed by the VAC-HET and finally the CTRL group.
Fig. 1. Picture of liquid cyclonic air collector. Liquid cyclonic air collector assembled for air sample collection (left). Disassembled liquid cyclonic air collector where the propeller and collection vessel are shown (right).
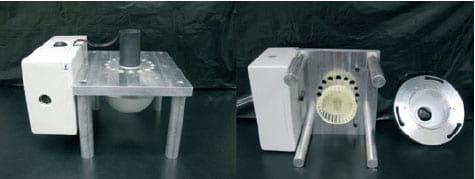
Air sampling procedures were collected by pouring 10 ml of minimum essential media (MEM) supplemented with 4% bovine serum albumin into the cyclonic collector collection vessel. The collector was hung from an aluminium pole 80 cm away from the ground and 1.45 m away from the wall and allowed to run for 30 min. The pigs did not have direct contact with the air sampling device. After collection, approximately 5 ml of MEM was removed from the collection vessel using a sterile syringe (Tyco-Healthcare, Kendall Monoject, Mansfield, MA, USA) and stored in sterile 15-ml polystyrene tubes (Sarstedt, Newton, NC, USA). The collector was then disinfected with alkyl dimethyl benzyl ammonium chloride spray (Lysol, Reckitt Benckiser, Wayne, NJ, USA), rinsed with water and dried with paper towels (Kim wipes, Kimberly-Clark, Roswell, GA, USA). After disinfection, the collection vessel and turbine were swabbed for PCR testing.
Diagnostic assays
All procedures were conducted at the University of Minnesota. Serum samples were tested by HI (Pedersen, 2008) and ELISA (IDEXX Flock ChekTM AI MultiSScreen; IDEXX Laboratories, Westbrook, ME, USA) (Ciacci- Zanella et al., 2010). Air samples, nasal and cyclonic collector swabs were tested by RRT-PCR (Spackman et al., 2002; Spackman and Suarez, 2008). Virus isolation was attempted on air samples collected between days 3 and 9 post-contact exposure on Madin–Darby canine kidney cells. Titration was attempted in VI positive air samples. Supernatants from air samples were titrated using tenfold serial dilutions and expressed as a log 10 TCID50/ ml calculated by the Spearman–Karber method.
Statistical analyses
All statistical procedures were conducted in SAS 9.2 (SAS Institute Inc., Cary, NC, USA). Differences were considered statistically significant at P < 0.05. HI and ELISA antibody titres comparison between groups was performed through analysis of variance. Fisher's exact test was used to assess the relationship between treatment and detection of influenza A virus in air samples by either RRT-PCR or virus isolation. Logistic regression was used to investigate whether there was a relationship between the numbers of RRT-PCR positive pigs and the ability to detect virus in the air. Relationship between the immune status of the pig and the presence of clinical signs was assessed by logistic regression repeated measures.
Results
Serum samples from pigs born to vaccinated sows collected before arrival to the isolation facilities were positive by HI and ELISA, and samples from pigs born to unvaccinated pigs were negative. HI titres against the challenge strain differed significantly between the VAC-HOM and the VAC-HET groups but not between the VAC-HET and the CTRL group (Table 1). A significant difference was detected on the ELISA titres between the pigs born to vaccinated sows and the pigs born to non-vaccinated sows. Nasal swabs from all three groups were RRT-PCR negative for influenza virus on arrival.
All three seeder pigs became infected with influenza A after challenge. A total of 330 nasal swabs were collected from all individual pigs from 0 days post-contact (DPC) until the end of the study period. All seeder pigs tested positive for influenza during the first three DPC. Besides the seeder pigs, six positive swabs were detected in the control group and none in both the VAC-HOM and VAC-HET groups on three DPC. From four DPC onwards, the number of positive swabs increased in both the CRTL and the VAC-HET group until 5 and 6, respectively, when they reached the maximum number of positive individual results (Fig. 2). Clinical signs in all three groups were mild and there were no significant differences between groups (P > 0.05).
Table 1. Geometric means of the reciprocal hemagglutination inhibition (HI) against the challenge virus and ELISA serologic results from pigs born to vaccinated and non-vaccinated sows before contact exposure
Fig. 2. Percentage of influenza A virus RRT-1 PCR nasal swab positive pigs by day post contact-exposure in each group (seeder pigs are excluded from the graph).
A total of 31 air samples were collected from each treatment group. In the VAC-HOM group, there were no positive air samples; however, one sample was classified as suspect at six DPC by RRT-PCR (Table 2). A total of 3.2% and 6.4% of the samples were positive in the VACHET and the CTRL groups, respectively. There were 19.3% samples considered suspect in the VAC-HET group and 9.6% in the CTRL group. There were no statistically significant differences between influenza A virus detection in the air across treatment groups and the number of either positive RRT-PCR or virus isolation positive air samples.
Influenza virus was isolated and titrated from six air samples. Four of the samples originated from the VACHET group with a virus titre of 3.2 x 102 TCID50/ml. Two of these samples were classified as suspect by RRTPCR at six DPC, one was positive by RRT-PCR at eight DPC and another was negative at eight DPC by RRTPCR. The other two titrated samples were from air samples from the CTRL group. The sample at seven DPC which tested positive by RRT-PCR had a virus titre of 3.2 x 102 TCID50/ml, whereas the sample that was classified as suspect on eight DPC had a virus titre of 1 x 102 TCID50/ml. All cyclonic collector post-disinfection swabs were RRT-PCR negative for influenza.
Owing to the low number of events (positive air samples) within groups, a logistic regression model to determine the relationship between positive pigs and detection of virus in the air could not generate estimates.
Discussion
Pre-farrow dam vaccination is widely used in swine to minimize the impact of influenza infections on breeding herds and neonatal pigs. The immune response generated in sows can be transferred to newborn piglets through ingestion of antibody-rich colostrum. The transfer of MDA can potentially protect neonatal pigs against influenza challenge including the reduction in clinical signs. However, it is not well understood whether pigs with MDA can be a source of infectious influenza aerosols when they become infected with influenza virus. Therefore, we conducted this study to evaluate whether pigs with MDA can generate infectious aerosols upon infection with influenza virus.
Results from our study indicate that pigs can be a source of infectious aerosols for influenza as virus was isolated from air samples. Furthermore, infectious aerosols could be generated by pigs with MDA without obvious clinical signs of influenza infection. Generation of infectious aerosols was more relevant in pigs with partial immunity (heterologous MDA) compared to pigs from the homologous MDA group in that this situation somewhat reflects current field conditions. The VAC-HET group is most likely to reflect field situations given the diversity of influenza infections in pigs. The presence of both influenza RNA and viable viral particles in air samples collected from recently weaned pigs with and without maternal immunity highlights the risk of aerosol transmission in neonatal pigs. In this study, pigs became infected and were able to generate infectious aerosols, suggesting that aerosol transmission can take place in partially immune breeding herds and weaned pig populations. Furthermore, pigs without obvious clinical signs of influenza infection could also generate infectious aerosols. Therefore, these results add one more piece of information to the complex ecology of influenza A virus in swine.
Individual pig results from the VAC-HOM group indicated that pigs were protected against influenza infection and virus transmission could only be detected in one contact pig. Protective immunity induced by vaccination can result in the reduction in nasal shedding and pneumonia (Bikour et al., 1996; Vincent et al., 2010) because of specific IgA and IgM limiting virus attachment and replication in the respiratory epithelial cells (Cox et al., 2004; Richt et al., 2006). In our study, pigs in the VAC-HOM group had significantly higher HI titres compared to the VAC-HET and CTRL groups. This may be the reason why there were no positive air samples identified in the VAC-HOM group. In contrast, there was active virus shedding in the CTRL and the VAC-HET group, which paralleled the detection of the virus in the air. Detection of the virus in the air was positive at six and seven DPC in the VAC-HET and CTRL groups, respectively, which also corresponded to the period of time when most (e.g. 80–100%) of the pigs were positive. Cross-reactive HI titres in the VAC-HET and the CTRL were significantly lower compared to the VAC-HOM group which most likely contributed to the susceptibility of influenza A virus infection and shedding of virus in aerosols in these groups.
The overall frequency of influenza RNA detection in air samples in this study is low compared to our previous experience (Corzo et al., 2011b). One possible explanation is the bodyweight and age of the pigs used in each study. Pigs in this study were 3 weeks old with an average weight at infection of 5.17 kg (SD 2.32) versus the pigs used in the previous study, which were 7 weeks old and weighed approximately 25 kg. Lung size is related to bodyweight and is considered a predictor of air exchange also known as tidal volume (TV). TV is the amount of air inspired and expired in one breath in a healthy animal (McDonell and Kerr, 2007). The TV for swine has been estimated to be 11 ml/kg (Riebold et al., 1995), suggesting that the volume of air expired by the 3-week-old pigs would be five times smaller than the air expired by 7- week-old pigs. On the basis of this, and assuming that 3- and 7-week-old pigs have similar virus titres in the lung (based on the same square area), the total amount of air containing viral particles aerosolized by 3-week-old pigs compared to 7-week-old pigs is predicted to be lower. Therefore, saturation of the air with influenza virus particles would be more efficient with larger pigs and therefore the likelihood of detection of viral particles in the air would also be higher in older pigs. Additionally, the level of virus replication in the lungs could also impact the level of virus found in aerosols. In this study, we did not attempt to quantify the level of virus replication in the lungs. Whether younger pigs would have similar or distinct virus replication rates in the lung compared to older pigs and whether that would have a significant effect on virus aerosol generation need to be further investigated.
Table 2. Number of positive and suspect RRT-PCR results and mean cycle threshold (Ct) values for nasal swabs and air samples, and number of virus isolation positive air samples and virus titres for each group throughout the study after introducing an influenza A virus infected pig (seeder pig) within each group
Alternatively, the virus could have been present in the air but under the detection limit of the cyclonic collector. Although the limit of detection for the cyclonic collector for influenza is considered low at 10 TCID50/ml (Corzo et al., 2011b), there might not have been enough virus accumulated in the air during the first few days post-contact to yield a positive result. This could be the result of specific housing conditions, rates of air change (e.g. 12–15 per hour in this study) or environmental conditions that may have influenced our ability to detect the virus in the air. In addition, we were not able to explore the relationship between the number of pigs shedding virus with detection of virus in the air most likely due to the low number of positive results available to fit the statistical model (Hosmer and Lemeshow, 2000).
Results from this study point towards the importance of aerosol transmission under field conditions. However, housing conditions in this study compared to field settings can be significantly different which may influence the direct applicability of these results. Pigs in this study were kept in small rooms with good air quality, constant ventilation rates and limited temperature oscillations that may not mimic field situations. Under field conditions, weaned pigs of similar age to the ones used in this study are kept in nursery or wean-to-finish facilities and may have lower or variable ventilation rates and higher densities, which may contribute to higher saturation rates of the air with infectious aerosols. In contrast, pigs in breeding herds prior to weaning are younger than the pigs used in this study, and overall piglet density in farrowing rooms will be lower, which may suggest that aerosol transmission might not be as relevant prior to weaning compared to post-weaning. Furthermore, under field conditions, pigs will have more variable levels of MDA which will also influence the degree of influenza susceptibility, virus replication, virus transmission and therefore the level of infectious aerosols generated. Nevertheless, further research is needed to assess the importance of aerosol transmission under field conditions and which factors contribute to it.
In summary, this study reports the detection of infectious airborne influenza A virus in air samples collected from weaned pigs with maternal immunity without obvious clinical signs. Despite the low number of positive air samples in pigs with partial immunity, this study highlights the potential for pigs with MDA to generate infectious aerosols. Airborne influenza A viral particles generated by weaned pigs or even suckling pigs can represent a risk of influenza transmission and further complicate the ecology of influenza virus and complexity of influenza transmission. More studies are needed to further our understanding on the effect of immunity on the generation of infectious aerosols and their role on influenza transmission under field conditions.
Acknowledgements
This study was partially funded by the Rapid Agricultural Response Fund, Minnesota Agricultural Experiment Station and the National Institute of Allergy and Infectious Diseases, National Institutes of Health, Department of Health and Human Services, under Contract No. HHSN266200700007C, and the Swine Disease Eradication Center at the University of Minnesota. Its contents are solely the responsibility of the authors and do not necessarily represent the official views of the NIH.
References
Allerson, M., and M. Torremorell, 2010: The epidemiology of influenza virus in sow farms: a case report. Proceedings of Allen D. Leman Conference, pp. 172, St. Paul, MN, USA.
Allerson, M., M. Torremorell, J. Deen, M. Gramer, H. S. Joo, and A. Romagosa, 2011: The impact of maternally derived immunity (MDI) on influenza virus transmission in neonatal pig populations. Proceedings of Allen D Leman Conference, pp. 69–70, St. Paul, MN, USA.
Bikour, M. H., E. Cornaglia, and Y. Elazhary, 1996: Evaluation of a protective immunity induced by an inactivated influenza H3N2 vaccine after an intratracheal challenge of pigs. Can. J. Vet. Res. 60, 312–314.
Brown, I., 2000: The epidemiology and evolution of swine influenza in pigs. Vet. Microbiol. 74, 29–46.
Choi, Y. K., J. H. Lee, G. Erickson, S. M. Goyal, H. S. Joo, R. G. Webster, and R. J. Webby, 2004: H3N2 influenza virus transmission from swine to turkeys, United States. Emerg. Infect. Dis. 10, 2156–2160.
Ciacci-Zanella, J. R., A. L. Vincent, J. R. Prickett, S. M. Zimmerman, and J. J. Zimmerman, 2010: Detection of anti-influenza A nucleoprotein antibodies in pigs using a commercial influenza epitope-blocking enzyme-linked immunosorbent assay developed for avian species. J. Vet. Diagn. Invest. 22, 3–9.
Corzo, C. A., M. Gramer, D. Lauer, and P. R. Davies, 2011a: Prevalence and risk factors for H1N1 and H3N2 influenza A virus infections in Minnesota turkey premises. Avian Dis. In Press.
Corzo, C. A., A. Romagosa, M. Gramer, S. Dee, R. Morrison, and M. Torremorell, 2011b: Detection of influenza virus in air from artificially generated aerosols and experimentally infected pigs. Proceedings of American Association of Swine Veterinarians Conference. pp. 43–44, Phoenix, AZ, USA.
Cox, R. J., K. A. Brokstad, and P. Ogra, 2004: Influenza virus: immunity and vaccination strategies. Comparison of the immune response to inactivated and live, attenuated influenza vaccines. Scand. J. Immunol. 59, 1–15.
Hayden, F., and A. Croisier, 2005: Transmission of avian influenza viruses to and between humans. J. Infect. Dis. 192, 1311–1314.
Hosmer, D. W., and S. Lemeshow, 2000: Applied Logistic Regression, 2nd edn. John Wiley & Sons, Inc.,, USA.
Jung, T., C. Choi, H. K. Chung, J. Kim, W. S. Cho, K. Jung, and C. Chae, 2002: Herd-level seroprevalence of swine-influenza virus in Korea. Prev. Vet. Med. 53, 311–314.
Karasin, A. I., G. A. Anderson, and C. W. Olsen, 2000: Genetic characterization of an H1N2 influenza virus isolated from a pig in Indiana. J. Clin. Microbiol. 38, 2453–2456.
Kitikoon, P., D. Nilubol, B. J. Erickson, B. H. Janke, T. C. Hoover, S. A. Sornsen, and E. L. Thacker, 2006: The immune response and maternal antibody interference to a heterologous H1N1 swine influenza virus infection following vaccination. Vet. Immunol. Immunopathol. 112, 117–128.
Loeffen, W. L. A., P. P. Heinen, A. T. J. Bianchi, W. A. Hunneman, and J. H. M. Verheijden, 2003: Effect of maternally derived antibodies on the clinical signs and immune response in pigs after primary and secondary infection with an influenza H1N1 virus. Vet. Immunol. Immunopathol. 92, 23–35.
Loeffen, W. L. A., N. Stockhofe, E. Weesendorp, D. van Zoelen- Bos, R. Heutink, S. Quak, D. Goovaerts, J. G. M. Heldens, R. Maas, R. J. Moormann, and G. Koch, 2011: Efficacy of a pandemic (H1N1) 2009 virus vaccine in pigs against the pandemic influenza virus is superior to commercially available swine influenza vaccines. Vet. Microbiol. 152, 304– 314.
Lowen, A. C., S. Mubareka, T. M. Tumpey, A. Garcia-Sastre, and P. Palese, 2006: The guinea pig as a transmission model for human influenza viruses. Proc. Natl. Acad. Sci. USA. 103, 9988–9992.
Maes, D., H. Deluyker, M. Verdonck, F. Castryck, C. Miry, B. Vrijens, and A. De Kruf, 2000: Herd factors associated with the seroprevalences of four major respiratory pathogens in slaughter pigs from farrow-to-finish pig herds. Vet. Res. 31, 3.
Maldonado, J., K. Van Reeth, P. Riera, M. Sitja, N. Saubi, E. Espun˜a, and C. Artigas, 2006: Evidence of the concurrent circulation of H1N2, H1N1 and H3N2 influenza A viruses in densely populated pig areas in Spain. Vet. J. 172, 377– 381.
McDonell, W. N., and C. L. Kerr, 2007. Respiratory system. In: Tranquilli, W. J., J. C. Thurmon, and K. A. Grimm (eds), Lumb & Jones' Veterinary Anesthesia and Analgesia, 4th edn. pp. 117–151. Blackwell Publishing, Ames, IA, USA.
Munster, J. V., E. de Wit, J. M. A. van den Brand, S. Herfst, E. J. A. Schrauwen, T. M. Bestebroer, D. van de Vijver, C. A. Boucher, M. Koopmans, G. F. Rimmelzwaan, T. Kuiken, A. D. M. E. Osterhaus, and R. A. M. Fouchier, 2009: Pathogenesis and transmission of swine-origin 2009 A(H1N1) influenza virus in ferrets. Science 325, 481–483.
Myers, K., C. Olsen, and G. Gray, 2007: Cases of swine influenza in humans: a review of the literature. Clin. Infect. Dis. 44, 1084–1088.
National animal health monitoring system (NAHMS). 2007: References of swine health and health management practices in the United States, 2006. Part II. Available at http://www.aphis. usda.gov/animal_health/nahms/swine/downloads/ swine2006/Swine2006_dr_PartII.pdf (accessed May 25, 2011).
Olsen, C. W., I. H. Brown, B. C. Easterday, and K. Van Reeth, 2006: Swine Influenza. In: Straw, B. E., J. J. Zimmerman, S. D'Allaire, and D. J. Taylor (eds), Diseases of Swine, 9th edn. pp. 469–482. Blackwell Publishing, Ames, IA, USA.
Pedersen, J. C. 2008: Hemagglutination-inhibition test for avian influenza virus subtype identification and the detection and quantitation of serum antibodies to the avian influenza virus. Methods Mol. Biol. 436, 53–66.
Poljak, Z., C. E. Dewey, S. W. Martin, J. Christensen, S. Carman, and R. M. Friendship, 2008: Prevalence of and risk factors for influenza in southern Ontario swine herds in 2001 and 2003. Can. J. Vet. Res. 72, 7–17.
Richt, J. A., P. Lekcharoensuk, K. M. Lager, A. L. Vincent, C. M. Loiacono, B. H. Janke, W. H. Wu, K. J. Yoon, R. J. Webby, A. Solorzano, and A. Garcia-Sastre, 2006: Vaccination of pigs against swine influenza viruses by using an NS1-truncated modified live-virus vaccine. J. Virol. 80, 11009–11018.
Riebold, T. W., D. S. Geiser, and D. O. Goble 1995: Clinical techniques for food animal anesthesia. In: Large Animal Anesthesia Principles and Techniques, 2nd edn. pp. 140–173. Iowa State University Press, USA.
Spackman, E., and D. L. Suarez, 2008: Type A influenza virus detection and quantitation by real-time RT-PCR. Methods Mol. Biol. 436, 19–26.
Spackman, E., D. A. Senne, T. J. Myers, L. L. Bulaga, L. P. Garber, M. L. Perdue, K. Lohman, L. T. Daum, and D. L. Suarez, 2002: Development of a real-time reverse transcriptase PCR assay for type A influenza virus and the avian H5 and H7 hemagglutinin subtypes. J. Clin. Microbiol. 40, 3256–3260.
Tellier, R. 2009: Aerosol transmission of influenza A virus: a review of new studies. J. R. Soc. Interface 6, 783–790.
Thacker, E., and B. Janke, 2008: Swine influenza virus: zoonotic potential and vaccination strategies for the control of avian and swine influenzas. J. Infect. Dis. 197, 19–24.
Vannier, P. 1999: Infectious causes of abortion in swine. Reprod. Dom. Anim. 34, 367–376.
Vincent, A. L., J. R. Ciacci-Zanella, A. Lorusso, P. C. Gauger, E. L. Zanella, M. E. Kehrli Jr, B. H. Janke, and K. M. Lager, 2010: Efficacy of inactivated swine influenza virus vaccines against the 2009 A/H1N1 influenza virus in pigs. Vaccine 28, 2782–2787.
Yee, K. Y., T. E. Carpenter, T. B. Farver, and C. J. Cardona, 2009: An evaluation of transmission routes for low pathogenicity avian influenza virus among chickens sold in live bird markets. Virology 394, 19–27.
This article was originally published in 2012 Blackwell Verlag GmbH • Transboundary and Emerging Diseases.