1. Introduction
Wheat is susceptible to Fusarium head blight (FHB) world-wide which causes both yield and quality losses, in addition to contamination of harvested grain with mycotoxins, particularly zearalenone (ZEN) and type B trichothecenes such as deoxynivalenol (DON). There are strict regulations in place for maximum contamination levels of these two toxins to reduce accumulation in the human and animal food chains. Both mycotoxins can be produced pre- and post-harvest. Although Fusarium graminearum requires higher moisture content levels compared to other more xerotolerant or xerophilic spoilage fungi (e.g., Penicillium and Aspergillus species, respectively), delayed or inefficient drying and unsafe storage practices can allow fungal growth and potential mycotoxin accumulation.
Recent studies have shown that under conducive moisture contents and over a wide range of temperatures, F. graminearum colonisation of stored wheat grain can cause significant dry matter losses (DMLs) and result in DON and ZEN contamination exceeding the EU maximum limits for unprocessed cereals for human food consumption (DON: 1.750 and ZEN: 100 µgKg−1) [1] and animal feed (DON: 8.000 and ZEN: 2.000 µgKg−1) [2]. Overall, <1% DML can result in significant impacts on quality losses and toxin contamination exceeding the EU limits [3–5].
Stored cereals in silos represent an ecosystem in which there are significant interactions between the abiotic and biotic factors [6]. Indeed, Limay-Rios et al. [7] showed that the main sources for contamination of stored wheat in grain silos are at the top of the silo, where the inlet for grain filling is placed, and the outlet, where the grain is removed from the silo. These areas and the silo walls represent areas where higher moisture content can occur due to moisture migration, leading to increased contamination with mycotoxins.
However, there is very little information on how fungal growth is initiated and the way in which mycelial colonisation occurs, especially in three dimensions. Most previous studies have been conducted by measuring colonisation in two directions or dimensions only [8,9]. However, in reality, filamentous fungi such as F. graminearum grow in stored grain in three dimensions extending their mycelium in all directions to obtain nutrients from the rich wheat substrate. Du et al. [10] and Vidal-Diez de Ulzurrun et al. [11] recently reported novel lattice-based models for a three dimensional study of fungal growth, but there are no publications that have examined Fusarium colonisation in stored cereal grain.
Measurement of ergosterol, fungal biomass and colony diameter have been positively correlated on agar media previously suggesting that the three parameters may be useful for primary modelling [12–14]. Colony diameter is extensively used to assess fungal growth. However, in aged colonies (>30 days) ergosterol did not increase exponentially, suggesting that despite the colony expansion in terms of area, there was a decrease in the rate of ergosterol accumulation [12]. Similarly, fungal diameter measurements may not be a perfect indicator of fungal biomass, especially in aging colonies where biomass may increase despite limitation in actual colony extension [13]. Other studies have used ergosterol content as a measure of the overall mould contamination levels of commodities and related this to safe or unsafe storage conditions [15,16]. In addition, the use of ergosterol was found to be an effective biochemical marker for early indications of fungal contamination as well as of predicted DON contamination levels in stored cereals [17,18].
However, few studies, if any, have examined the colonisation of cereals by a mycotoxigenic species in three dimensions to try to understand the growth kinetics and the relationship between growth and toxin production. There is also a lack of knowledge of how temporal fungal colonisation occurs in a 3D matrix such as stored grains via the intergranular spaces, and how this may be affected by the initial inoculum position or the prevailing environmental factors.
The objectives of this study were to examine the kinetics of 3D spatial colonisation of stored wheat grain by F. graminearum and the accumulation of DON and ZEN when inoculated in different positions and under different water availabilities in laboratory microcosm storage systems. This allowed information to be obtained on (a) spatial patterns of colonisation depending on the different initial inoculum positions, (b) the changes in respiration and associated DMLs, (c) quantification of fungal biomass, using ergosterol, (d) the temporal DON and ZEN mycotoxin accumulation during colonisation and (e) the correlation between these different biological and physical parameters.
2. Materials and Methods
2.1. Fungal Isolate and Spores Preparation
F. graminearum strain Fg 08/111 isolated from wheat in the UK was cultivated on V8 vegetable juice plates (V8®, 175 mL; CaCO3, 3 g; ZnSO4·7H2O, 0.01g; CuSO4·5H2O, 0.005g; agar, 20 g·L−1) and incubated in the dark at 25 °C for ten days to obtain heavily sporulating cultures [19]. Following incubation, 3 mL of sterile Tween 80® (0.05%) was poured on the Petri plates previously incubated and then gently scratched with a sterile Drigalsky’s spatula. The liquid was transferred to a second Petri plate containing fungi and the procedure repeated with the remaining 4 Petri plates. The resulting liquid was recovered and collected in a sterile falcon tube. After homogenizing, the number of spores per mL was determined using a Thoma counting chamber (Celeromics, Grenoble, France) and the final concentration adjusted to 1 × 104 spores·mL−1 in sterile water + Tween 80 (0.005%).
2.2. Grain Preparation, Inoculation and Incubation
Dry wheat grain (0.71 water activity (aw), 13.5 % moisture content (m.c.)) was irradiated with 12–15 kGy (Synergy Health, Swindon, UK) to reduce microbial contaminants while maintaining germinative capacity of the grain, and stored at 4 °C until use. The grain aw levels were modified to 0.95 and 0.97 by adding known amounts of sterile water and reference to an adsorption curve for this batch of wheat grain, which was detailed in Garcia-Cela et al. [20], and verified using the Aqualab 4TE aw meter.
Square jars of 136 mL capacity (Pattersons Glass Ltd., Grimsby, UK) (see supplementary Figure S1) with a 9 mm diameter hole in the jar lid, which was closed with a cotton wool plug to allow gaseous exchange while avoiding contamination, were autoclaved at 121 °C for 20 min. Single grains inoculated with a drop of 5 µL of the 104 spores mL−1 suspension (approx. 50 spores per grain) were placed in distinct positions to provide initial inoculum of F. graminearum for initiating growth in the different positions within the jars: (a) top-centre, (b) bottom-side and (c) bottom-centre. For the bottom inoculations, an 8 µL drop of organic clear nail polish was used to fix the grain on the jar bottom. Fixed grains were inoculated with the stated spore solution drop and 1 min later, the remaining grains were added to the jar. For the top-centre position, the spore solution drop was placed over a single grain in the centre of the top layer of grains. In all cases, 15 jars per treatment combination were used, including 3 samples without F. graminearum inoculated per aw tested.
Inoculated jars were incubated at 25 °C for 10 days. To maintain atmospheric equilibrium relative humidity, jars were placed in food storage containers (10 L volume) containing beakers of glycerol–water solution at the treatment aw level (6 × 100 mL per container). Glycerol–water solutions were renewed once (day 5) during the experimental period.
2.3. Colonisation Pattern Assessment
Fungal colonisation of the wheat grain was followed by recording the superficial mycelial extension visible through the six sides of the square clear jars (supplementary Figure S2). Clear labels with a mesh of 0.5 × 0.5 cm were aligned and pasted on the bottom and sides of the jars. To avoid external contamination of the grains, jar lids were opened under sterile conditions and a plastic sheet with the same mesh was aligned on the top. Visual fungal colonisation was followed every 24 h until the grain was fully colonised.
The colonisation data were used to compute volumetric colonisation (cm3) by F. graminearum from the three inoculation treatment positions. In two dimensional studies, fungal growth is known to expand in circular colonies following a radial pattern [21]. In the present study, we expanded the approach assuming that mycelial colonisation followed an ellipsoidal shape before being constrained by the cubic shape of the jar. The detailed procedure followed to compute the volumetric colonisation is presented in Appendix A.
2.4. Respiration Determination and Dry Matter Loss Estimation
Every 24 h, samples were sealed for 1 h at 25 °C with injection lids that contained polytetrafluoroethylene (PTFE)/silicone septa (20 mm diameter × 3.175 mm thickness) to allow for CO2 accumulation in the headspace. Headspace volumes of jars containing grain at 0.95 or 0.97 aw were, respectively, 90.67 and 88.33 mL. In both growth conditions, 5 mL of headspace air were withdrawn with a syringe and directly inserted into the Gas Chromatograph (GC) for CO2 analysis. An Agilent 6890N Network GC (Agilent Technologies, Cheshire, UK) with a Thermal Conductivity Detector and helium as a carrier gas was used. A Chromosorb 103 packed column was usedand the data were analysed using Agilent Chemstation Software (Agilent Technologies, Cheshire, UK).
GC was calibrated with a standard gas bottle of 10.18% CO2 and 2% O2 in nitrogen (British Oxygen Company, Guilford, Surrey, UK). F. graminearum CO2 production was obtained by subtracting the sample respiration of a blank culture that had not been inoculated with the fungal species. The percentages of CO2 production were used to calculate the Respiration (R) in mg CO2 (kg·h −1), total cumulative production CO2 and total DML as described in Mylona and Magan [3].
2.5. Mycotoxins Extraction and Analysis
Before mycotoxin extraction, samples were dried at 60 °C for 24 h and then milled in a laboratory blender (Waring Commercial, Christian, UK). Mycotoxins accumulation of three replicate samples per combination of inoculation position and aw on alternate days (days 2, 4, 6, 8 and 10) were extracted by adding 500 µL of acetonitrile:water:formic acid (79:20.9:0.1, v:v:v) to 100 (± 10) mg of milled wheat and agitated for 90 min at 300 rpm at 25 °C on a rotary shaker (miniShaker VWR, Leighton Buzzard, UK). Then, samples were centrifuged for 10 min at 22,600 g (Centrifuge 5417S Eppendorf, Stevenage, UK), and 1 or 3 µL of the supernatant was injected into an Exion LC series HPLC linked to a 6500+ qTRAP-MS system in Electrospray Ionisation (ESI) mode (Sciex Technologies, Warrington, UK). An ACE 3-C18 column (2.1 × 100 mm, 3 µm particle size; Hichrom) with guard cartridge (4 × 3 mm, Gemini, Agilent) was conditioned at 40 °C. The elution gradient used water:acetic acid (v:v, 99:1, solvent A) and methanol:acetic acid (v:v, 99:1) (solvent B), both supplemented with 5 mM ammonium acetate. The 15 min-long gradient included: 0 min, 90% A; 0–2.0 min, 90–60% A; 2.0–10.0 min, 0% A and 10.0–11.50 min, 0% A; 11.50−12.0 min, 90% A; 12.00−15.0 min, 90% A at a 0.3 mL·min−1 flow rate. 10 msec of dwell time per daughter ion (2 per metabolites) was used in an unscheduled Multiple Reaction Monitoring (MRM) in both positive and negative mode using the parameters listed in Table 1.
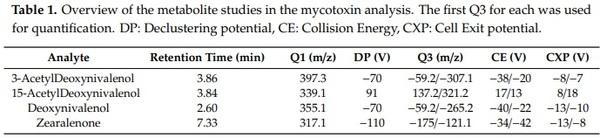
The source conditions used included: Curtain gas 40%, Collision Gas Medium, IonSpray voltage 4500 V/ 5500 V, Temperature 400 °C, both ion sources gas at 60 psi, with a 10 V Entrance Potential for all compounds. Data acquisition was conducted with Analyst® Data Acquisition version 1.6.3, and quantification through MultiQuant™ version 3.0.3.
The analyte recovery, the limit of detection (LOD) and the limit of quantification (LOQ) were calculated using recommendations stated elsewhere [22]. The calculated recoveries were used as correction factors to calculate the absolute concentration of the analytes. The LODs were 0.26 and 2.29 ng·g−1 and the LOQs 0.85 and 7.63 ng·g−1 for ZEN and DON, respectively
2.6. Ergosterol Analysis
The replicates and treatments were oven-dried at 60 °C for 48 h, milled in a laboratory blender (Waring Commercial, Christian, UK), homogenised, and the ergosterol content analysed using an adaptation of the rapid ultrasonic method developed previously [23]. This involved adding 2 mL of deionised water to a 5 g of sample. After 15 min, 10 mL methanol:ethanol (v:v, 4:1) was added and samples were stored at 4 °C for 2 h. Then, 20 mL hexane:propan-2-ol (v:v, 98:2) was added to each sample and they were ultrasonicated at 150 W in an ice bath with water for 3 min 20 s. After 30 s, 2 mL of the supernatant was centrifuged at 7000× g for 10 min, from which 1.5 mL was used for HPLC analysis.
The HPLC system was a Shimadzu Class-VP, which consisted of SCL-10Avp controller, SPD-10Avp detector and LC-10ADvp pump (Shimadzu Corporation, Japan). An amount of 100 µL was injected into a Lichrosorb Phenomenex column (250 × 4.6 mm, 10 µm) at 35 °C. Run time for samples was 15 min with ergosterol being detected at about 9.20 min. The flow rate of the mobile phase (hexane:propan-2-ol; v:v, 98:2) was 1.4 mL·min−1.
Calibration standards of 5, 25, 50, 100 and 150 µg·g−1 ergosterol in hexane:propan-2-ol (v:v, 98:2) were used as an external calibration. A general recovery test was conducted from spiked samples using 4 replicates. The procedure was the same as explained above, with two changes: (a) 1 mL of internal standard was added after the deionised water and samples were allowed to stand for 15 min, (b) 19 mL of hexane:propan-2-ol (v:v, 98:2) was added instead of 20 mL. Average recovery rates for 50, 100, 500 and 1000 µg·g−1 ergosterol from wheat grain matrix were 89.18 ± 14.44%, 104.35 ± 12.37%, 106.37 ± 9.81% and 94.41 ± 5.97%, respectively.
2.7. Data Analysis
Data were analysed with JMP Pro (JMP®, version 14. SAS Institute, Inc., Cary, NC, USA). All data were assessed for normality and homoscedasticity. Growth rate data, DML, respiration and ergosterol, were normally distributed, homoscedastic and independent. Therefore, data sets were analysed using two-way Analysis of Variance (ANOVA) for the determination of the significance between aw treatments and inoculation position. Then, Student0 s t- or Tukey0 s HSD test were used to identify significant differences between groups. Mycotoxins accumulation data were not normally distributed, therefore a Kruskal–Wallis test for the determination of the significance between aw treatments and inoculation position was undertaken. Then, non-parametric comparisons for each pair was conducted using the Wilcoxon test. The statistical relationship between variables was assessed by Pearson or Spearman correlations. A signification level of 5% was assumed for all statistical analysis.
The “fit_growthmodel” function of the “growthrates” R package [24] was used to fit the colonisation data to a two-phase linear model of the form:
where Ct is the colonisation (cm2 or cm3) at time t (days), λ a lag phase with 0 colonisation (days) and Rc the colonisation rate (cm2·day−1 or cm3·day−1).
3. Results
3.1. Fungal Colonisation
F. graminearum showed a rapid colonisation rate at both tested aw levels with all of the wheat grain visually completely colonised after 5–6 days. A comparative example of the recorded superficial growth showed distinct colonisation patterns of F. graminearum depending on the initial inoculum position (Figure 1). Superficial colonisation data were well described using a two-phase linear model (Figure 2) providing estimates of the lag time prior to colonisation and the superficial colonisation rate (cm2·day−1) shown in Table 2. The lag phase length prior to superficial colonisation was shown to be significantly affected by the inoculation position but not by aw, with mean lag phase times higher for the bottom-side position (2.97 ± 0.22 h) than the bottom-centre (2.92 ± 0.31 h) and top-centre (2.94 ± 0.32 h) positions. No significant differences (α = 0.05) in the colonisation rate were detected among treatments. As a consequence of this increased lag time, the bottom-side inoculations were able to completely colonise the grain surface only by day 6 of the experiment. For the other inoculum positions (top-centre and bottom-centre) this occurred after 5 days (Figure 3a,b).
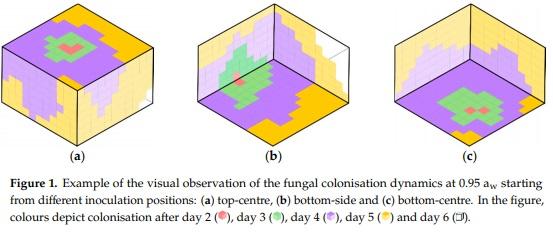
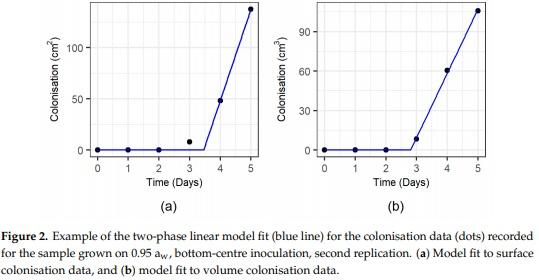
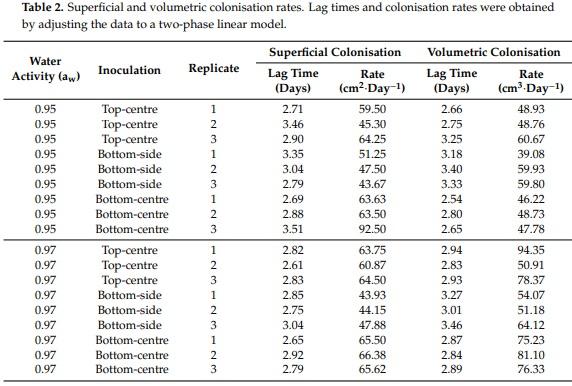
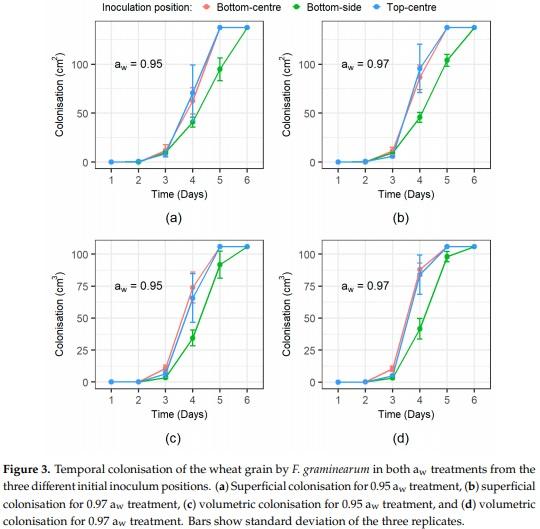
Volumetric fungal colonisation (cm3) was obtained from the visible colonisation assuming that the fungal growth of the grain was consistent with a partial ellipsoidal expansion of the mycelia constrained by the cubic volume of the jar (see Appendix A). Volumetric colonisation (Figure 3b,c) was also well adjusted to a simple two-phase linear model, obtaining colonisation lag times and volumetric colonisation rate (cm3·day−1) estimates shown in Table 2. The length of the lag phase was inoculation having an extended lag period (3.28 ± 0.16 h) distinguishable from that of the top-centre (2.89 ± 0.21 h) and bottom-centre (2.76 ± 0.14 h) inoculum positions. The volumetric colonisation rate were significantly affected by aw, and was more rapid at 0.97 aw (69.52 ± 15.24 cm3/day) than at 0.95 aw (51.10 ± 7.42 cm3·day−1).
3.2. Indirect Indicators of Fungal Growth
3.2.1. Fungal Respiration Dynamics
Cumulative fungal respiration for the 10 day experimental period is shown in Figure 4a,b. In all inoculation positions and aw treatments, respiration increased over time as the F. graminearum mycelia colonised the wheat grain. The accumulated respiration at the end of the colonisation process (days 6 for bottom-side, and day 5 for top-centre and bottom centre inoculations) was significantly affected by the initial inoculation position (p-value = 0.022). Fungal colonisation from the bottom-side proceeded more slowly but produced more CO2 (4.96 ± 0.84 g·CO2·kg−1) than the top-centre (3.83 ± 0.79 g·CO2·kg−1) and bottom-centre (3.23 ± 0.52 g·CO2·kg−1). d bottom-centre (3.23 ± 0.52 g·CO2·kg−1). Bottom-side respiration was significantly higher (α = 0.05) than the bottom-centre respiration. However, these two treatments were indistinguishable from the top-centre cumulative respiration.
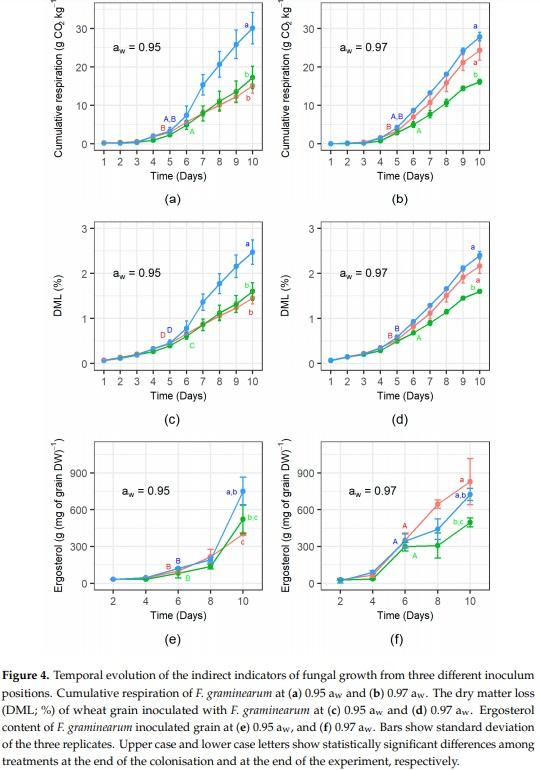
The ANOVA analysis at the end of the experiment (day 10), suggested a significant effect of the inoculation treatment position (p-value < 0.001) and an interaction amongst the inoculation positions and aw factors (p-value = 0.003). The 0.95 aw top-centre, the 0.97 aw top-centre, and 0.97 aw bottom-centre treatments were similar. The other treatments: 0.95 aw bottom-side, 0.97 aw bottom-side, and 0.95 aw bottom-centre, were similar but significantly lower than the previous group (Figure 4a,b).
3.2.2. Dry Matter Loss Dynamics
The DML followed a similar temporal increase at both aw levels depending on the inoculum position (Figure 4c,d) and reflected the respiration rates (see Figure 4a,b). However, there was a more linear increase earlier than that for the respiration rate. At the end of the colonisation period (days 6 for bottom-side, and day 5 for top-centre and bottom-centre inoculations) the DML was significantly affected by aw levels (p-value = 0.001) and the inoculation position (p-value = 0.002). The DMLs were higher at 0.97 aw than in the slightly drier 0.95 aw treatment (0.60 ± 0.07 vs. 0.51 ± 0.09% respectively). As observed for respiration, grain colonisation initiated from the bottom-side position proceeded more slowly and generated more DML (0.64 ± 0.06%) by the time the grain was completely colonised than the top-centre (0.53 ± 0.08%) and bottom-centre (0.49 ± 0.06%) treatments.
The ANOVA analysis at the end of the experiment (day 10) suggested a significant effect of both aw (p-value = 0.018) and inoculation position (p-value < 0.0001) treatments, and for the interaction between these two factors (p-value = 0.003). The 0.95 aw top-centre, 0.97 aw top-centre and 0.97 aw bottom-centre treatments were very similar. The remaining treatments again grouped together and had significantly lower DMLs than the other group of treatments.
3.2.3. Ergosterol Production Dynamics
During the colonisation process, ergosterol content of all of the treatment combinations appeared to be mainly driven by the storage aw (Figure 4e,f). By day 6, close to the end of the colonisation of the wheat grain, aw had a significant effect (p-value<0.001) on ergosterol content, although was interestingly not influenced by the inoculation position (p-value = 0.07). Overall, there was a significantly higher ergosterol content at 0.97 aw (331.56 ± 50.76 g mg−1) than at 0.95 aw (100.97 ± 27 g·mg−1). At the end of the experimental period, ergosterol content was statistically affected by the aw (p-value = 0.027), inoculation position (p-value = 0.005) and the interaction between these two factors (p-value = 0.004). The 0.97 aw bottom-centre produced a higher ergosterol content than the bottom-side inoculation and 0.95 aw bottom-centreinoculation. Top-centre inoculations were very similar statistically to the 0.97 aw bottom-centre treatment and the bottom-side inoculations. Similarly, bottom-side inoculations could not be distinguished from the 0.95 aw bottom-centre treatment.
3.3. Mycotoxin Production Dynamics
Mycotoxin production was significantly impacted by the aw treatment (p-value < 0.0001 and p-value = 0.0331 for DON and ZEN, respectively) with higher production occurring at 0.97 aw. DON production could be quantified from day 4 for the wetter 0.97 aw (Figure 5b) condition and from day 6 in the drier 0.95 aw treatment (Figure 5a). For ZEN production, this was predominantly found from day 6 onwards in both aw treatments (Figure 5). ZEN increased markedly by the end of the experimental period (10 days). Unlike for colonisation of the wheat grain, the inoculum position did not significantly impact either DON or ZEN production.
3.4. Correlation between Experimental Measures
3.4.1. Correlation between Fungal Respiration and Ergosterol Content
There was a significant correlation between ergosterol content and cumulative respiration data for F. graminearum inoculation in wheat grain. Spearman rank order correlation suggested a strong positive correlation between these two variables (Table 3). When considering individual aw treatments the correlation was stronger during the colonisation period but this decreased when both aw levels are considered together. The overall correlation coefficient among water activities for the length of the experiment was 88.58%
3.4.2. Correlation between Mycotoxin Contamination Levels and Indicators of Colonisation
Correlations between the growth indicators used in this study and the mycotoxin production is shown in Table 4. For DON, all of the indicators used (i.e., volume, ergosterol and cumulative DML) showed a positive correlation. The correlations ranged from 0.8149 to 0.9528 and were dependent on the aw treatment, with higher correlations at 0.95 aw than 0.97 aw. The cumulative DML and the ergosterol content were found to be the indicators most correlated to DON contamination level.
For ZEN, all indicators except for the volume of growth obtained at 0.95 aw correlated well with the mycotoxin content. The average correlation ranged from 0.4150 to 0.8193. Higher correlation factors were observed at 0.97 aw when compared to 0.95 aw. The best correlated indicators for ZEN were the cumulative DML at 0.97 aw and both cumulative DML and Ergosterol at 0.95 aw.
Overall, DON and ZEN production showed a good correlation at 0.95 aw (0.6417) and 0.97 aw (0.7604).
4. Discussion
This study found differences in the colonisation patterns from different initial inoculum positions for a mycotoxigenic filamentous fungus such as F. graminearum. The inoculation position affected the length of the lag phase but not the actual rates of mycelial colonisation. This suggests that the geometry of the mycelia expanding through the rich nutritional matrix had an effect on the fungal expansion via the intergranular spaces. To our knowledge, this is the first attempt to follow and model the volumetric colonisation of such stored commodities. An assumption was made that colonisation followed an ellipsoidal front. Volumetric data obtained was adjusted assuming a two phase linear model. Being a non-linear transformation, one would expect the non-constrained volumetric colonisation to be better fitted by a non-linear relationship. Nonetheless, in our conditions, this could be explained by the fact that the colonisation was constrained by the finite cubic shape of the storage jars shortly after initial growth. Alternative techniques for examining the volume of colonised grains could include visualisation techniques that allow exploration of internal fungal growth, such as resin impregnation and thin slicing [25], or non-invasive Computed Tomography (CT). Visualisation of the fungal colonisation using X-ray CT is currently challenging due to the similar absorption properties of fungi and organic matter, although it may be possible for fungi accumulating minerals and colonisation in wood [26].
Unlike the superficial colonisation rate, volumetric colonisation rates were significantly different between aw levels. Significant differences in the volumetric colonisation pattern between aw treatments were found in this study that followed similar (pseudo) 2D fungal growth patterns where mycelial colonisation by F. graminearum of layers of wheat grain was more rapid in wetter grain [8,9]. However, the differences in colonisation patterns in the former studies only examined superficial colonisation rates and did not take account of a volumetric 3D grain matrix with multiple grain layers as opposed to a single layer of grain. The exploratory nature of this filamentous fungus is thus able to more effectively utilise the nutrients from the wheat grain in different directions.
Analysis of the cumulated respiration at the end of the colonisation period suggested that (i) fungi required more energy to colonise the grain when the colonisation was slower, and (ii) that the aw levels tested do not significantly affect this process. Differences in the grain colonisation dynamics from different initial inoculum positions may be related to the ability of the hyphae to grow into the intergranular spaces to colonise other wheat grains and produce the necessary extracellular enzymes to exploit the rich nutritional substrate effectively. This may have resulted in the differences observed in respiration dynamics with faster colonisation (bottom-centre and top-centre) than other inoculum positions. The low respiration levels found in the bottom-centre colonisation at 0.95 aw may thus have been due to lower oxygen levels in the intergranular spaces and the more stressful water availability treatment. Overall, the present results show that fungal colonisation rates can be accurately estimated by the amount of CO2 produced in different stored cereals [3,5,27,28].
In the present study, fungal respiration rates and DML obtained by CO2 measurements supported the colonisation data sets. Fungal respiration rates have been correlated with DML, as fungal growth produces CO2 due to the oxidation of carbohydrates and production of water vapour and heat during aerobic respiration [29]. DML has been previously used as a quality indicator of stored grains [29,30]. Therefore, the differences among contamination points reported in the present study suggests that contamination source might have an impact on quality losses in a storage silo.
In our study, ergosterol as a fungal biomass measurement was shown to be affected by both aw level and the inoculation position. Considering that the analysis performed at day 6 did not allow the comparison of the ergosterol amounts at the exact end of the colonisation, the statistical analyses showed the effect of inoculum position on the fungal biomass produced based on ergosterol. This supports the hypothesis that the fungal biomass composition changes depending on the rates of colonisation. A higher ergosterol production in the wetter conditions (0.97 aw) was probably due to the increased utilisation of the carbohydrates in the wheat grain by the F. graminearum. Overall, the present results are similar to the ergosterol levels found in wheat cultivars infected by F. graminearum by Stuper-Szablewska et al. [31], although they used a different ergosterol analysis method. Despite the absence of a widely accepted analysis method for ergosterol as a biomass indicator in cereals, it has been directly correlated with fungal colonisation of cereals and DON contamination [18,32,33]. However, as it is a destructive method it cannot be performed in real time. Thus, few attempts have been made in food science to develop a high-recovery method for this fungal biomass indicator in food commodities [34–36].
This study showed that respiration rates and ergosterol content have a highly significant positive correlation. Previously, DML has been successfully correlated with Fusarium mycotoxin levels [3,5] and can be calculated from measured respiration rates. Therefore, the correlation between respiration rate and ergosterol found suggests that further research should be conducted to examine the relationship between ergosterol content, respiration rates and mycotoxin contamination levels.
Finally, mycotoxin production (both DON and ZEN) was found to be unaffected by the inoculation position but was higher in the wetter growth condition. The higher DON and ZEN production at 0.97 aw when compared to 0.95 aw was due to the effect of the relative water stress the mycotoxigenic species was exposed to. F. graminearum is more sensitive to drier conditions [9]. The present study showed earlier production of DON at day 4 at 0.97 aw compared to day 6 at 0.95 aw. Our results also showed ZEN production starting at day 6 independently of the aw tested and an increase in production by day 10. Our study also showed that production of these mycotoxins occurred within 6 days, with the inoculum position having no effect on relative DON and ZEN production patterns. Previously, strains of F. graminearum from Argentina were shown to produce higher amounts of DON at 0.97 aw (43 ng·g−1) compared to none at 0.95 aw in wheat gains after 7 days incubation [8]. They also found that at 0.95 aw production of DON only occurred after 14 days. Ezekiel et al. [37] monitored the production of ZEN in wheat at 0.95 aw every 6 days and showed that production only occurred after day 12 followed by a steady increase in ZEN production.
The lack of effect of the inoculation position in mycotoxin content may be related to the fact that the whole sample was homogenized before the mycotoxin extraction. One previous report showed larger toxin clusters and stronger spatial autocorrelation in the outer grain layers in a silo, in which the higher humidity and more favourable oxygen availability resulted in better fungal development, while the presence of toxins in deeper locations in the stored grain was related to the influence of gravity [38]. This highlights that more information is needed about the differences between outer and inner layers within a large grain mass in terms of fungal growth and toxin contamination.
This study showed a strong correlation between cumulative DML and DON production found at both aw levels (0.9220 at 0.95 aw; 0.8669 at 0.97 aw), similar to that of Mylona et al. [4] who found a 0.9572 spearman correlation between DML and DON production on wheat at three different aw levels (0.89, 0.94, 0.97 aw) at 15 to 30 °C. The present study also showed a significant correlation between ZEN production and DML (0.5971 at 0.95 aw; 0.8193 at 0.97 aw). Similar correlations were observed by Garcia-Cela et al. [5] and Mylona et al. [4]. Both correlations for DON and ZEN production provide effective information to develop post-harvest management tools to be integrated for improved Decision Support Systems (DSS).
5. Conclusions
In this study, the behaviour of F. graminearum in stored wheat in terms of grain colonisation and mycotoxin production (DON and ZEN) was evaluated in a 3D volume for a period of ten days. Primary inoculum position affected the initial growth significantly and therefore the colonised grain. Modern silos are currently monitored at different spatial levels, consequently, spatial modelling could be used to predict the level of risk. Respiration and DML indicators seem to be as reliable as ergosterol measurements (to indicate fungal colonisation) but with the advantage that they can be monitored in real-time. Thus, to perform efficient silo management, different approaches must be tailored to each of the spatial areas covered by the sensors in which the alert was detected.
The results of this study revealed that understanding the fungal growth pattern and the diffusion of multiple mycotoxins is essential for the development of accurate predictive models that can support effective post-harvest management of grain. This is critical as grain is traded on a wet weight basis and very slight changes in the moisture can lead to an increase in the activity of mycotoxigenic spoilage moulds and mycotoxin contamination.
This article was originally published in Microorganisms 2020, 8, 1170; doi:10.3390/microorganisms8081170. This is an Open Access article distributed under the terms and conditions of the Creative Commons Attribution (CC BY) license (http://creativecommons.org/licenses/by/4.0/).