Summary
Dietary lipids and fatty acids are not only fundamental in determining animal performance, but also determine the eating qualities of animal products. Several methods have been used to quantify fatty acid metabolism but most involve expensive in vitro approaches that are not suitable for most laboratories. Furthermore, there is considerable variation between methods with regard to enzyme activity, which makes comparison of results between studies difficult. The recently developed whole-body fatty acid balance method (WBFABM) is a simple and reliable in vivo method for assessing fatty acid metabolism, including rates of liponeogenesis and de novo fatty acid production, β-oxidation of fatty acids and bioconversion (elongation and desaturation) of fatty acids to long-chain polyunsaturated fatty acids. Initially developed for implementation with a fish model, the WBFABM has proven to be a simple and effective method that can be used in any laboratory equipped with a gas chromatography unit. Since its development, it has been used in several farmed finfish feeding trials and in broiler chicken feeding trials. The WBFABM is currently used at research institutions worldwide and its use is increasing in popularity among animal scientists. With this method, it is possible to track the fate of individual dietary fatty acids within the body. The WBFABM could contribute significantly to information generated by animal feeding trials.
Introduction
Lipids are ubiquitous in cells and fulfil a variety of functions essential for life. They are present in the body as preferential sources of available energy, structural components of biomembranes, precursors of eicosanoids, hormones and vitamin D, enzyme cofactors and carriers of fat-soluble vitamins. Consequently, the lipid components of diets are important for the promotion of growth, health, reproduction and other body functions. Of the nutrients, lipids contain the highest amount of energy per unit weight (39.5 kJ/g, roughly double that that of proteins and carbohydrates). Therefore, their catabolism via mitochondrial or peroxisomal β-oxidation to produce energy in the form of ATP plays a major role in nutrition. With the exception of free cholesterol, all classes of lipid contain one or more fatty acid molecules. Fatty acids can be grouped into three major classes according to their degree of unsaturation: saturated fatty acids (SFAs), monounsaturated fatty acids (MUFAs) and polyunsaturated fatty acids (PUFAs). Furthermore, PUFAs are often sub-classed as omega-3 (n-3) or omega-6 (n-6) PUFAs according to whether the first double bond is located at the third or sixth carbon, respectively, and the use of the term, long-chain polyunsaturated fatty acid (LC-PUFA), is becoming increasingly common.
Lipids and fatty acids in animal nutrition
To adapt to the aquatic environment and its trophic chain, which is particularly rich in proteins and lipids and very poor in carbohydrates, fish metabolism has evolved to utilise lipids preferentially, specifically fatty acids, as dietary sources of energy. Consequently, in fish feed, lipid sources are the most effective raw materials for an optimal balance of protein and energy (Bell and Koppe, 2010). Although terrestrial animals such as poultry use carbohydrates efficiently for energy production, lipids are a highly concentrated energy source and are useful for feed formulation (McDonald et al., 1988). Dietary lipids are important for both terrestrial and aquatic animals because they contain essential fatty acids. Essential fatty acids are defined as fatty acids indispensable for normal functioning of the organism that cannot be synthesised de novo by the organism itself and consequently must be obtained from the diet. No terrestrial or aquatic farmed animals, as is the case with all vertebrates, are able to produce n-3 and n-6 PUFAs de novo. The bioactive forms of these fatty acids include the LC-PUFA, eicosapentaenoic acid (20:5n-3), docosahexaenoic acid (22:6n-3) and arachidonic acid (20:4n-6). The majority of farmed animals are able to produce 20:5n-3, 22:6n-3 and 20:4n-6 from α-linolenic acid (18:3n-3) and linoleic acid (18:2n-6). 18:3n-3 and 18:2n-6 are produced by plants and algae and are therefore normally termed basic essential fatty acids and should always be provided in adequate amounts in the feed (McDonald et al., 1988; Bell and Koppe, 2010). However, from an etymological viewpoint, this definition of essential fatty acids is not totally correct because i) it has been shown that 18:3n-3 and 18:2n-6 can be synthesised in vivo by bioconversion of shorter fatty acids such as 16:3n-3 and 16:2n-6, respectively, and ii) from a physiological point of view, the truly "essential" fatty acids are the LC-PUFAs. For a comprehensive discussion of this subject, the reader is referred to Cunnane (2003).
The lipid nutrition and fatty acid metabolism of fish is of significant interest to the global aquaculture sector because of environmental and economic issues pertaining to the use of fish oil in aquaculture feed (Turchini et al., 2009). The challenge for fish nutritionists is to reduce the utilisation of fish oil in aquafeed formulations while ensuring that an appropriate amount of n-3 LCPUFAs are present in the final product. Similarly, fat metabolism in terrestrial farm animals and the fatty acid composition of their products and tissues is the subject of much ongoing research (Poureslami et al., 2010a). Control of fat deposition is of great economic importance in meat producing animals. In addition, the fatty acid composition of intramuscular and subcutaneous lipids has nutritional and technological implications, e.g., meat could be a valuable source of n-3 LC-PUFA for humans and the unsaturated to saturated fatty acid ratio of animal fats affects their suitability for meat processing. Total body fat content and fatty acid composition are determined by genetic and environmental factors. Of the environmental factors, diet has by far the largest effect. In all farm animal species, body fatty acids are derived from dietary uptake, de novo synthesis and bioconversion.
Thus, paradoxically, whereas fish nutritionists are currently focusing their research effort on the effects of reducing the dietary supply of n-3 LC-PUFA, terrestrial farm animal nutritionists are interested in understanding the potential to increase the dietary supply of n-3 LCPUFA. In both cases, a reliable in vivo method for assessing fatty acid metabolism is paramount.
Assessing fatty acid metabolism in animals
After digestion, dietary fatty acids are absorbed and transported to various organs, where they may be incorporated into triacylglycerols and stored as reserve energy, incorporated into membrane phospholipids or oxidised to produce metabolic energy. Dietary fatty acids may be used as is or they may be bioconverted by alternating chain elongation and desaturation reactions catalysed by fatty acid elongase and desaturase enzymes. Numerous studies have been performed to investigate the effects of source and content of dietary fat and the duration of feeding fat on the fatty acid composition of various tissues. In many of these studies, inferences about fatty acid metabolism were made from analyses of the fatty acid composition of tissue samples after controlled feeding and slaughter experiments. More fundamental studies have used in vitro and in vivo approaches with labelled fatty acids (Brown, 2005).
A variety of methods exists for the assessment of fatty acid metabolism ex vivo and in vivo. The most widely used method involves isolating whole cells (e.g., hepatocytes) or tissue microsomes and incubating them with labelled fatty acids (e.g., [1–14C]18:2 n-6 or [1–14C]18:3 n-3). During the past decade, the abundant use of ex vivo methods has significantly increased our understanding of some aspects of lipid metabolism. However, these analytical methods require expensive and sophisticated equipment that is unavailable at the majority of research institutions. Furthermore, these methods differ greatly depending on the application and some do not take important factors such as fatty acid-binding proteins into account or measure flux through pathways such as β-oxidation (Brown, 2005). In vivo methods employ a whole-body approach and enable estimation of an organism''''s overall capacity to metabolize fatty acids within the context of an integrated system (Cunnane and Anderson, 1997; Turchini et al., 2007). In vivo methods are practical, relatively simple, require routine laboratory techniques and can be applied to almost all organisms. Surprisingly, few studies have relied on the whole body balance approach for investigating fatty acid metabolism even though the serial slaughter technique and whole carcass homogenization followed by analysis of the gross chemical composition has been regularly applied to study changes in body composition during growth and development.
The whole body fatty acid balance method
The whole-body fatty acid balance method (WBFABM) was initially proposed by Cunnane''''s group (Chen and Cunnane, 1993; Cunnane and Yang, 1995; Cunnane and Anderson, 1997) and was subsequently developed further and refined by Turchini et al. (2007) and Turchini and Francis (2009). The method involves quantification of the initial and final fatty acid composition of the wholebody and quantification of the net intake of dietary fatty acids in a feeding trial. It can be used in conjunction with a very short feeding trial that results in minimal or no weight gain or even weight loss (Chen and Cunnane, 1993). However, it has been suggested that when applied to farmed animals, for which individual variation is greater than for laboratory animals, the feeding trial should be of sufficient duration to accommodate a change in weight to enable accurate quantification of fatty acid metabolism (Turchini et al., 2007). As the method is fundamentally a mass balance analysis, it is possible to increase the sensitivity of the measurement by increasing differences between variables. It is crucial that the feed intake is measured accurately. A total collection of faeces or a corresponding digestibility estimation is essential for quantifying the net intake of fatty acids. The analyses required for this method are: initial and final body weight, initial and final fatty acid composition of the whole body, total feed intake, fatty acid composition of the diet and fatty acid digestibility or composition of the faeces.
If the primary objective of the investigation is to study the conversion of C18PUFA to LC-PUFA in a species that has a limited bioconversion capability, the absence of LC-PUFA from the diet is preferred. However, this condition is not obligatory, and is of less concern for species with relatively efficient bioconversion capabilities, such as fish and poultry, as is discussed later in this review.
The WBFABM involves four steps. The first step is to determine the partitioning of dietary fatty acids between excretion, accumulation, appearance and disappearance, as described by Cunnane and Anderson (1997). The second step involves a backwards computation along the three main fatty acid pathways, the n-3 PUFA pathway, the n-6 PUFA pathway and the SFA and MUFA pathway. Briefly, the amount of fatty acids represented by a specific metabolic pathway is converted from mg to mmol of fatty acids appearing or disappearing. The number of mmol of longer chain or more unsaturated fatty acids appearing is subtracted from the number of mmol of the previous fatty acid in the specific fatty acid pathway. Estimation of the fate (elongation, desaturation or oxidation) of each fatty acid can therefore be computed accordingly to its specific metabolic pathway. At this point (the third step of the method), it is possible to quantify the amount of an individual fatty acid used for β-oxidation and de novo biosynthesis. The activities of specific enzymes (elongase, Δ-5, Δ-6 and Δ-9 desaturase) and the rates of β-oxidation and de novo biosynthesis are estimated in the fourth and final step of the method. Enzyme activity is then expressed as mmol (or μmol) of product per gram of body weight (average body weight) per day and is conventionally reported as "apparent in vivo enzyme activity" to distinguish it from enzymatic activity measured using in vitro methods. In the following sections (and as depicted in Figure 1), examples of how WBFABM results are interpreted and used to optimise feed efficiency and product quality are presented.
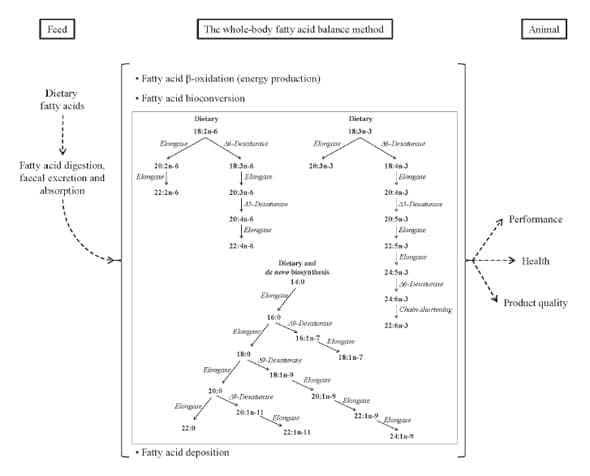
Figure 1. The whole-body fatty acid balance method. Fatty acid concentrations in feed, faeces and the whole body are analysed in a feeding trial and used to calculate deposition, bioconversion and β-oxidation of fatty acids.
Examples of results obtained using fish
The WBFABM has been used to study the Murray cod (Maccullochella peelii peelii), rainbow trout (Onchorhynchus mykiss), Nile tilapia (Oreochromis niloticus), red hybrid tilapia (Oreochromis sp.) and barramundi (Lates calcarifer) (Turchini et al., 2006; 2011; Francis et al., 2007; 2009a; 2009b; Turchini and Francis 2009; Alhazzaa et al., 2011; Senadheera et al., 2011; Teoh et al., 2011).
The first attempt to elucidate the fate of individual fatty acids using the WBFABM was reported by Turchini et al. (2006), who studied the effects of dietary canola oil and linseed oil on Murray cod. For the first time, this species was shown to be capable of fatty acid biosynthesis via the n-6 and n-3 pathways. In fish fed the canola oil diet, 54.4% of the 18:2n-6 consumed was accumulated, 38.5% was oxidized and 6.4% was elongated and desaturated to higher homologs. In fish fed the linseed oil diet, 52.9% of the 18:3n-3 consumed was accumulated, 37% was oxidized and 8.6% was elongated and desaturated. Murray cod also showed a preferential order of utilisation of C18 fatty acids for energy production (18:3n-3 > 18:2n-6 > 18:1n- 9). Moreover, it was demonstrated that an increase in dietary 18:3n-3 was directly responsible for increased desaturase activity and augmented saturated fatty acid accumulation in the body of the fish. The total elongase activity and total Δ6-desaturase activity was higher (P < 0.01 and P < 0.001, respectively) in fish fed the linseed oil diet compared with fish fed the canola oil diet. The presence of Δ5-desaturase activity could only be quantified in fish fed the linseed oil diet, whereas in fish fed the canola oil diet, there was no accumulation of any fatty acid after the Δ5-desaturation step in the elongation and desaturation pathway. In fish fed the linseed oil diet, Δ6-desaturase activity (14.34 ± 0.37 μmol/g/d) was significantly higher than elongase activity (8.56 ± 0.99 μmol/g/d), and both were significantly higher than Δ-5 desaturase activity (0.75 ± 0.54 μmol/g/d).
These authors subsequently used the method to investigate potential methods for alleviating the global shortage of fish oil. Francis et al. (2007) investigated modulation of fatty acid metabolism in Murray cod using graded increments (0–100%) of a blended vegetable oil formulated to mimic the fatty acid profile of fish oil. The WBFABM revealed that the rate of accumulation of both n-3 and n-6 LC-PUFAs decreased as the substitution of fish oil with vegetable oil approached 100%. With respect to the overall balance of individual fatty acids, there was an accumulation and significant appearance of 18:3n-6, 20:3n-6, 18:4n-3 and 20:4n-3 despite negligible dietary intakes of these fatty acids. Levels of n-6 and n-3 LC-PUFAs decreased as substitution of fish oil with vegetable oil approached 100%. The WBFABM revealed that although the balance of 20:4n- 6, 20:5n-3 and 22:6n-3 was negative in each of the treatments, the values became progressively closer to being positive as the level of vegetable oil substitution in the diets increased. Elongase and Δ-6 desaturase activity increased as vegetable oil inclusion increased; fish that received 100% vegetable oil had the highest elongase and Δ-6 desaturase activities. The WBFABM was used to evaluate the presence of a feedback mechanism affecting desaturase and elongase activities in Murray cod fed graded dietary levels of C18 PUFA with a constant ratio of 18:2n-6/18:3n-3 (1/1) (Francis et al., 2009a). This study demonstrated a greater degree of apparent enzyme activity (Δ-6 desaturase activity) in fish fed diets containing high concentrations of C18 PUFAs. In fish receiving high concentrations of C18 PUFA, Δ-6 desaturase reacted with 18:3n-3 to a greater extent than with 18:2n-6. As the C18 PUFA concentration was reduced, the substrate preference of Δ-6 desaturase changed from 18:3n-3 to 18:2n-6. This indicates that Δ-6 desaturase has a greater affinity for 18:3n-3 than for 18:2n-6 when C18PUFA are provided in abundance. However, a higher affinity towards 18:2n-6 over 18:3n- 3 was recorded when substrate intake was low. Thus, the authors suggested that, in Murray cod, the primary LC-PUFA requirement that needed to be fulfilled was represented by 20:4n-6, above that for 20:5n-3 and 22:6n-3. Once this requirement has been met, and if sufficient substrate is available, the affinity of Δ-6 desaturase for the n-3 substrate increases. Therefore, the authors concluded that the absolute quantity of Δ-6 desaturated fatty acids was proportional to substrate availability, but the maximal efficiency of the Δ-6 desaturase enzyme was attained at average substrate availability. Senadheera et al. (2011) took this approach one step further by investigating the role of dietary 18:2n-6 and 18:3n-3 on LC-PUFA bioconversion in the same species. However, this study evaluated the effects of different 18:2n-6 to 18:3n-3 ratios while maintaining a constant level of C18PUFA. The results of this study indicated that 18:3n-3 was more actively β-oxidised and bioconverted than 18:2n-6, which was deposited to a greater degree than 18:3n-3. Interestingly, 18:2n-6 was β-oxidised at a constant rate (~36% of net intake), but 18:3n-3 was β-oxidised at a rate proportional to its dietary supply. In synchrony with the increasing dietary 18:2n-6 availability, an increase in the in vivo apparent Δ-6 desaturation of 18:2n-6 was recorded. On the other hand, when dietary 18:3n-3 availability increased, a higher in vivo apparent Δ-6 desaturation of 18:3n-3 was recorded. This data provided a clear indication that Δ-6 desaturase enzyme activity is substrate dependent. This study revealed that Δ-6 desaturase activity for 18:3n-3 peaked at a substrate level of 3.2186 μmol/g/d, whereas that for 18:2n-6 peaked at a substrate level of 4.1512 μmol/g/d. It was concluded that dietary inclusion of 18:3n-3 and 18:2n-6 at levels greater than required to attain these maxima would be wasteful in terms of n-3 LC-PUFA bioconversion. Additionally, it was also shown that Δ-6 desaturase enzyme had a 3.2-fold greater affinity for 18:3n-3 than for 18:2n-6. The WBFABM was also used to assess the effects of alternated feeding schedules in Murray cod (Francis et al., 2009b) and to identify a new phenomenon termed the "omega-3 sparing effect" of MUFA in farmed fish (Turchini et al., 2011). It was shown that an abundant dietary supply of MUFA, and to lesser extent SFA, spares n-3 LC-PUFA from oxidation for energy production and increases their deposition.
Turchini and Francis (2009) used the WBFABM to compare the fates of individual fatty acids in rainbow trout fed fish oil- or linseed oil-based diets. Similar to the results for Murray cod, much of the 18:3n- 3 provided by the linseed oil diet was oxidised for energy production. Despite the appearance of fatty acids because of Δ-6 and Δ-5 desaturation, whole-body concentrations of 20:5n-3 and 22:6n-3 were 2- and 3-fold less, respectively, for the linseed oil diet than for the fish oil diet. In general, there was a greater net disappearance for surplus fatty acids. Therefore, in fish receiving the fish oil diet, a large proportion of 20:5n-3 was used for energy production and a small amount was converted to 22:6n-3 and deposited. For the first time, these authors applied the WBFABM to record a net appearance of SFA and MUFA resulting from de novo production of fatty acids. Rainbow trout receiving the linseed oil diet exhibited a net appearance of 18:4n-3, which was 62-fold higher than concentrations observed in fish that received the fish oil diet, in which it was preferentially oxidised.
Teoh et al. (2011) investigated fatty acid metabolism responses in Nile tilapia and red hybrid tilapia to a vegetable oil blend formulated to mimic the major fatty acid classes of fish oil. This study demonstrated an increase in β-oxidation, Δ-6 desaturase activity and Δ-5 desaturase activity in both species of fish receiving the vegetable oil based diet, whereas no Δ-5 desaturase activity was apparent in the n-3 pathway for the fish oil treatment. Regarding the n-6 conversion pathway, fish were shown to be capable of bioconversion of 18:2n-6 to n-6 LC-PUFA. From a dietary formulation viewpoint, the WBFABM proved useful in showing that a higher level of SFA and MUFA would be beneficial because of the high rate of liponeogenesis in both species.
Alhazza et al. (2011) used the WBFABM with barramundi fed echium (rich in 18:4n-3) or a canola oil diets to test the hypothesis that consumption of a diet rich in 18:4n-3 increases n-3 LC-PUFA biosynthesis. They demonstrated that barramundi are capable of desaturating and elongating fatty acids to higher homologs, but not to the degree originally anticipated, and that they have low rates of conversion of fatty acids to n-3 LC-PUFA. Fish receiving the 18:4n-3-rich diet did not exhibit Δ-6 desaturase activity.
Examples of results obtained with livestock species
The WBFABM is rarely used with livestock species because of the high cost and labour associated with homogenization of whole carcasses, particularly those of the larger species of livestock. Therefore, it is not surprising that the few studies in which this method has been used have involved growing chickens and pigs. Poureslami et al. (2010a,b) used the WBFABM to study the effects of dietary fat source, age and sex on fatty acid metabolism in broiler chickens. Crespo and Esteve- Garcia (2002) also analysed whole body fatty acids in broilers fed different dietary fat sources to determine the efficiencies of energy, fat, protein and fatty acid deposition, but restricted their calculations to the main classes of fatty acids. Kloareg et al. (2005, 2007) and Duran-Montgé et al. (2010) applied a whole body fatty acid balance approach to growing pigs. Methodological differences exist between these studies, making it difficult to compare some of the results.
Poureslami et al. (2010a) compared various lipid sources (palm fat, soybean oil, linseed oil or fish oil), which were included at a level of 3% in a basal diet containing 5% palm fat, for female and male broilers. The experiment was conducted in two phases: when the broilers were 7–21 days of age and when they were 21–42 days of age, and thus encompassed the entire fattening period. Sex had no effect on fatty acid metabolism, and age had a minor effect compared with that of dietary fat source. Accumulation of 18:2n-6 was higher than that of 18:3n-3, whereas bioconversion to longer chain metabolites and oxidation were lower for 18:2n-6 than for 18:3n-3. Accumulation of 18:3n-3 was 63% of net intake and was not affected by the diet. Accumulation of 18:2n-6 was higher with the linseed oil diet than with the other diets (85% vs 74% of net intake, respectively). Bioconversion of 18:2n-6 was lower for the fish oil diet than for the plant oil diets (1% vs 3–5% of net intake, respectively), confirming the well-known inhibitory effect of long chain n-3 PUFA on n-6 PUFA metabolism as a result of competition for the same desaturase enzymes. 18:3n-3 bio-conversions were similar for the palm fat and soybean oil diets and were greater than that for the linseed oil diet (9% vs 6% of net intake, respectively). β-oxidation of 18:2n-6 was lower for the linseed oil diet than for the other diets (11% vs 23% of net intake, respectively), whereas β-oxidation of 18:3n-3 was higher for the fish oil diet than for the other diets (42% vs 27% of net intake, respectively). Fish oil suppressed apparent elongase and desaturase activity in terms of μmol/g/d, whereas a higher dietary supply of 18:3n-3 and 18:2n-6 enhanced apparent elongase and desaturase activity in terms of the PUFA involved in the n-3 and n-6 pathways, respectively.
Although this experiment was not designed for investigating SFA and MUFA metabolism, these authors also analysed their data for this purpose (Poureslami et al., 2010b). The accumulation (percentage of net intake and de novo production) of SFA and MUFA was lower for the palm fat diet than for the other diets (86 vs 97%, respectively). Conversely, β-oxidation was higher for the palm fat diet than for the other diets (14% vs 3% of net intake and de novo production, respectively). A mean of 33% of the total SFA and MUFA accumulated in the body was elongated, and 14% was Δ-9 desaturated to a longer chain or to more unsaturated metabolites. A lower proportion of total SFA and MUFA were elongated and desaturated with the palm fat and fish oil diets compared with the soybean and linseed oil diets. Total in vivo apparent elongase activity decreased exponentially in relation to the net intake of SFA and MUFA. Somewhat surprisingly, total in vivo apparent Δ-9 desaturase activity was not affected by dietary treatment or age. The total de novo production and β-oxidation rates of SFA and MUFA had negative and positive curvilinear relationships, respectively, with net intakes of SFA and MUFA, respectively.
Bazinet et al. (2003) used the WBFABM (as described by Cunnane and Anderson, 1997) to evaluate the oxidation of 18:2n-6 and 18:3n-3 in piglets subjected to a segregated early weaning (SEW) system or a non-segregated weaning system (NSW). In the SEW system, pigs are weaned when they are 10–14 days old instead of at 21 days old and are housed in a cleaner environment compared with NSW system, which is commonly used in commercial units. SEW pigs are reported to eat more and grow faster, and it was suggested that this is due to reduced antigen exposure, i.e., to less demand on their immune system (Bazinet et al., 2003). Pigs were fed a control diet (n-6/n-3 = 21.3) or a high 18:3n-3 diet (n-6/n-3 = 2.5) and were weaned either at 14 days old into a SEW nursery or at 21 days old into a conventional NSW nursery. NSW pigs had 15–25% lower carcass 18:2n-6 content (expressed as a percentage) and 20–30% lower carcass 18:3n-3 content at 49 days of age. Between 35 and 49 days of age, the NSW pigs had higher whole-body oxidation of 18:2n-6 (40–120%) and 18:3n-3 (30–80%) than SEW pigs. The high-18:3n-3 diet decreased whole-body oxidation of 18:2n-6 by 73% and that of 18:3n-3 by 63% in NSW pigs. The authors concluded that moderately cleaner housing (SEW) significantly decreases 18:2n-6 and 18:3n-3 oxidation in pigs.
Kloareg et al. (2005) fed a diet containing 3.6% fat (no added fat) to young pigs from 24 kg to 65 kg live weight at 23 °C or 30 °C and at four feeding levels to investigate the effect of high ambient temperature and feed restriction on fatty acid deposition. Kloareg et al. (2007) fed a diet containing 1.5% soybean oil (total fat content = 4.4%) to two genotypes and sexes of slaughter pigs from 90 kg to 150 kg live weight. In both studies, digestibility values for dietary fatty acids were obtained from the literature and it was assumed that 70% of dietary non-essential fatty acids were deposited as is. This assumption was based on the observed 70% deposition vs 30% net oxidation of total n-6 PUFA. Although the authors acknowledged that the extent of oxidation depends on fatty acid chain length and saturation, they argued that the assumption of a constant oxidation rate for all fatty acids had little effect on the outcome of their studies because of the low dietary fat content.
Kloareg et al. (2005) estimated a deposition rate of 69% for total n-6 PUFA (deposition of 18:2n-6 and conversion to 20:3n-6 and 20:4n-6), and thus an oxidation rate of 31%. The corresponding values for 18:3n-3 were 48% for deposition and 52% for oxidation (long chain n-3 PUFA were not measured). These authors used a data analysis model to partition de novo synthesized fatty acids and showed that the quantitatively most important transformations were elongation of 16:0 to 18:0 and desaturation of 18:0 to 18:1. The effects of temperature and feeding level on the partitioning of de novo synthesized fatty acids were also observed. Kloareg et al. (2007) observed much lower values for accumulation of PUFA in the body (31% and 40% of digestible n-6 and n-3 PUFA, respectively) and concomitantly higher values for PUFA that could not be accounted for and were assumed to have been oxidized or converted to non-fatty acid metabolites. They hypothesized that the large difference in oxidation rate between their studies might be related to differences in the stage of development of the animals and in dietary 18:2n-6 and 18:3n-3 content. In chickens, it has been shown that a higher dietary PUFA supply increases the oxidation of these fatty acids (Crespo and Esteve- Garcia, 2002). In the study of Kloareg et al. (2007), of the 40% of dietary 18:3n-3 that was recovered in the body, more than one-third was deposited as 20:5n-3, 22:5n-3 and 22:6n-3. This suggests that conversion of 18:3n-3 to these long chain metabolites is more efficient in pigs than in humans (Brenna et al., 2009). A similar conclusion was made by Poureslami et al. (2010a) for broiler chickens.
Duran-Montgé et al. (2010) fed pigs a diet with a very low level of fat or one of six fat-supplemented diets differing in fat source. Ileal digestibility values had been determined in a previous study. They calculated 18:2n-6 deposition rates of 65–73% for diets rich in this fatty acid. Deposition rates for 18:3n-3 were 63–64%. Diets rich in PUFA resulted in lower PUFA deposition rates and deposition generally decreased as the degree of unsaturation increased. The observed deposition rates for the long-chain n-3 PUFAs, 20:5n-3 and 22:6n- 3 were 48% and 49%, respectively, for a diet containing fish oil.
Conclusions
As dietary lipids and fatty acids affect animal performance and the eating qualities of animal products, in vivo estimations of fatty acid metabolism are of paramount importance for optimal feed formulation and management. The WBFABM is a simple, but very powerful analytical tool that is capable of tracking the individual fate of each dietary fatty acid within the body of a growing animal. In theory, the method can be applied to any organism, requiring little more than a gas chromatography unit for fatty acid analysis, appropriate experimental protocols and elementary calculations. The WBFABM is a simple, effective and easily implemented method that can contribute significantly to information obtained from animal feeding trials.
Acknowledgements
Giovanni Turchini's contribution to this article was made while holding an Australian Research Fellowship from the Australian Research Council (Project DP1093570) "Triggering the dormant capacity of fish to make omega 3 fatty acids" and this support is gratefully acknowledged. The views expressed herein are those of the authors and are not necessarily those of the Australian Research Council.
This paper was presented at the Recent Advances in Animal Nutrition (RAAN)- Australia. Vol 18, 2011.