INTRODUCTION
Inflammation is an important, highly conserved, innate immune system response. In birds, like in mammals, the inflammatory response is activated by various pathogen- or damage-associated molecular patterns through their interaction with cellular and soluble pattern-recognition receptors (Juul-Madsen et al., 2014; Kaspers and Kaiser, 2014; Abbas et al., 2018). For example, lipopolysaccharide (LPS; endotoxin), a cell wall component of gram-negative bacteria, is a potent stimulator of inflammation. It initiates inflammatory activities via Toll-like receptor 4 expressed on the cell surface of leukocytes, such as monocytes/macrophages and heterophils (Dil and Qureshi, 2002; Kogut et al., 2005). This ligand–receptor interaction activates macrophages to produce and release vasoactive mediators, cytokines (e.g., interleukin (IL)-1β, IL-6, tumor necrosis factor-alpha; TNF-α), and chemokines (e.g., IL-8) (Klasing, 1991; Dil and Qureshi, 2002; Juul-Madsen et al., 2014; Kaspers and Kaiser, 2014; Rohde et al., 2018). While these effects of LPS have been clearly established in avian cell cultures, the inflammatory processes initiated by LPS at the site of inflammation are less well understood, although individual studies in chickens suggest activities initiated by LPS parallel those described for mammals (Abbas et al., 2018).
Briefly, inflammation is a process that occurs in vascularized tissue. Injection of LPS activates local macrophages and other leukocytes to produce cytokines, chemokines, and vasoactive factors. These factors then lead to vascular changes that are instrumental in recruitment and extravascular accumulation of phagocytes and plasma proteins from the blood, as well as activation of the recruited cells (Nair 1973; Klasing, 1991). The first type of leukocytes to accumulate at the site of LPSinduced inflammation is heterophils (the avian counterpart of mammalian neutrophils) which infiltrate rapidly (within hours) and return to the baseline levels 24 to 48 h later. Monocytes are also recruited from the blood to the site of LPS injection, but at a slower pace than heterophils (Chansoriya et al., 1993; More Bayona et al., 2017). In tissues, monocytes differentiate into macrophages, and, once activated, exhibit a broader spectrum of activities than heterophils, including enhanced phagocytosis, production of powerful microbicidal factors, as well as pro-inflammatory and anti-inflammatory cytokines, tissue repair, and resolution of the inflammatory response (Klasing, 1991; Kaiser et al., 2012; Byrne, 2016; Lieboldt et al., 2017; More Bayona et al., 2017; Abbas et al., 2018).
In commercial broiler production, broiler health depends greatly on maternal antibodies, in ovo or day-of-hatch vaccinations, and innate immune system defenses, given that the short production period of broilers is predominantly during the development phase of their adaptive immune system (Hamal et al., 2006; Simon et al., 2014). While maternal immunity and antibiotic feed supplements support their health, the demand for products from antibiotic-free poultry production poses threats to both broiler health and production efficiency (Centner, 2016). Hence, focus on optimizing innate immunity in broilers is a natural approach toward a healthy and safe product. The study and evaluation of innate immune system responses, such as the inflammatory response, are complicated by the fact that the response takes place in tissues. Although leukocytes and plasma proteins that accumulate in affected tissues originate from the blood, the complex interplay of vascular changes, molecular signaling cascades, and local cellular activities that are key to effective containment and elimination of microbial infection cannot be ascertained from a blood sample alone (Smith et al., 1975; Chansoriya et al., 1993; More Bayona et al., 2017).
The skin and it derivatives, from wattles, wing web, foot pads, dewlap, and skin pouches to, more recently, the pulp of growing feathers (GF), have been used as cutaneous test tissues to study cellular responses to intradermally (i.d.) injected test materials in avian species (Jankovic et al., 1963; Klesius et al., 1977; Awadhiya et al., 1981; Taylor et al., 1986; Corrier, 1990; Chansoriya et al., 1993; Scott and Siopes, 1994; Erf and Ramachandran, 2016). Of these, the GF is the only cutaneous tissue for which repeated sampling of the same individual is minimally invasive; that is, conducting biopsy by plucking the loosely attached injected GF from its follicle is less invasive than sampling the vasculature. Laboratory analysis of the collected GF tissue provides novel insight into in vivo activities in response to injected materials (Erf, 2012; Erf and Ramachandran, 2016; Erf et al., 2017; Sullivan and Erf, 2017). Moreover, blood can also be sampled along with injected GF, affording simultaneous insight into local and systemic activities initiated by i.d. injection of test material, over time, in the same individual (Byrne, 2016; Erf et al., 2017).
The GF and blood dual-window approach has the potential to emerge as an important, balanced system to study and evaluate immune system responses in chickens. However, thus far, the GF cutaneous test has only been used in egg-type chickens. In the context of optimizing natural defenses in commercial broilers, adaptation of this test for use in broilers promises to yield an efficient and balanced approach to study and evaluate innate immune system activities such as inflammation.
The objective of this study was to use the GF and blood dual-window approach for the first time in commercial broilers and to examine the broiler’s local and systemic inflammatory responses initiated by GF injection of LPS which is an established and relevant immunostimulator in chickens (Bowen et al., 2009; De Boever et al., 2009). Multiple GF were injected i.d. with LPS, followed by simultaneous, periodic sampling of GF and blood in the same individuals. As early events in the inflammatory response are the most critical for the immune system to effectively outpace microbial infections (Robinson et al., 2019), the focus of this study was on the early, acute phase of the inflammatory response to LPS (6 and 24 h after GF injection). Laboratory measurements included determination of leukocyte infiltration profiles and associated changes in blood cell profiles, local reactive-oxygen-species (ROS) generation and antioxidant enzyme activities, as well as local expression patterns of inflammation-related target genes.
MATERIALS AND METHODS
Experimental Animals and Diets
The University of Arkansas Institutional Animal Care and Use Committee (IACUC) approved all protocols and procedures involving animals used in this trial (IACUC Protocol # 16054). Cobb MVx500 straight run broiler chicks (n = 55) from a commercial hatchery were obtained on day of hatch. Chicks were placed in a temperature- and light-controlled biosecure room at the Arkansas Experiment Station’s Poultry Health Laboratory, Division of Agriculture, University of Arkansas, Fayetteville, on wood shavings litter with feed and water available ad libitum. All chicks were penned together in a 0.9 m x 1.8 m floor pen until 7 d of age when 2 males and 2 females were randomly assigned to one of 12 0.9 m x 0.9 m pens. Lighting schedule and specific temperature targets were implemented and adjusted in accordance with management guidelines set forth by the primary breeder (Cobb-Vantress, 2015). The wellbeing of birds was checked daily. A common starter diet meeting the primary breeder nutrient recommendations was fed to chicks until 7 d after hatch. Thereafter, chicks were fed a basal corn-soybean meal–based grower diet formulated to meet primary breeder nutrient recommendations except for Cu, Zn, and Mn concentrations. Four experimental diets in which copper, zinc, and manganese were supplemented as either inorganic sulfate or hydroxychloride (IntelliBond, Micronutrients USA, LLC, Indianapolis, IN) forms and at 2 different concentrations (5 mg/kg Cu and 45 mg/kg of Zn and Mn, or 10 mg/kg Cu and 90 mg/kg of Zn and Mn) were fed for the remainder of the trial, with 3 pens per diet (12 pens total). The birds were fed the experimental different diets as part of another goal to determine the effect of the variation in trace mineral supplementation on the inflammatory response initiated in GF of broilers.
Initiation of a Local Inflammatory Response in the Dermis of Growing Feathers by Pulp Injection of Lipopolysaccharide
When the chicks were 16 d old, a row of 12 GF on each breast tract were plucked and allowed to regenerate for 18 d to yield rows of uniform GF for injection. When the chicks were 34 d of age, one male and one female chicken were randomly chosen from each of the 12 pens for GF injection with Salmonella typhimurium LPS (Sigma, Chemical Company, Saint Louis, MO). Specifically, the pulp of 8, 18-day-old regenerating GF on each breast feather tract was injected (i.d.) with 10 μL of 100 μg LPS/mL (1 μg/GF) in endotoxin-free Dulbecco’s PBS (Sigma) as previously described (Erf and Ramachandran, 2016; Erf et al., 2017; Sullivan and Erf, 2017). The mean BW ± SEM at time of GF injection was 2.044 ± 0.056 kg and 2.338 ± 0.041 kg for females and males, respectively; hence, the LPS dose was 7 to 8 μg per kg BW. The dose of 1 μg LPS per GF was previously determined in egg-type chickens to reliably result in a local inflammatory response that was accompanied by inflammation associated changes in the blood (Byrne, 2016). The pulp of GF of an additional 8 broilers (4 males and 4 females) was injected with 10 μL PBS to determine the local leukocyte infiltration response due to the injection, as this was not previously examined in broiler GF.
Sample Collection
Heparinized blood (2 mL) and 6 GF were collected before (0 h) and at 6 and 24 h after pulp injection of LPS from each of the 12 male and 12 female experimental animals. At each time point, 6 GF samples were collected; 2 GF were placed into a tube of ice-cold PBS and kept on ice until preparation of pulp cell suspensions, whereas the other 4 GF were placed inside an aluminum foil pouch, immediately flash-frozen in liquid nitrogen, and stored at −80°C for later analyses. Blood was collected from the wing vein using heparinized 3 mL syringes with 25 gauge x 1 inch needles (Becton Dickinson, Franklin Lakes, NJ). Care was taken to completely stop the bleeding before the bird was returned to its pen. Use of the left- and right-wing vein was alternated at different time points. One mL of blood was used for same-day automated hematology analysis (Wang et al., 2003) and the other mL of blood was used for plasma isolation. Plasma samples were stored at −80°C until use.
Preparation of Pulp Cell Suspensions, Immunofluorescent Staining
Pulp cell suspensions of the sampled GF were prepared as described previously (Erf and Ramachandran, 2016). Briefly, at each time point, the entire pulp of the 2 GF from each bird was pulled out of the sheath, placed in 1 mL PBS containing 0.1% collagenase (type IV, Life Technologies, Carlsbad, CA) and 0.1% dispase II (Boehringer Mannheim, Mannheim, Germany), and incubated at 40°C for 10 min. After incubation, the pulps were gently pushed through a 60-mm nylon mesh while adding ice-cold PBS. The pulp cell suspensions were washed twice in PBS at 250 x g for 4 min at 4°C and the pellet resuspended in a final volume of 0.5 mL with PBS. Cell suspensions were kept on ice until use for immunofluorescent staining or determination of ROS generation by kinetic fluorescence assay.
Immunofluorescent Staining of Pulp Cell Suspensions and Cell Population Analysis by Flow Cytometry
Pulp cell suspensions were immunofluorescently stained (Erf and Ramachandran, 2016) using fluorescently labeled mouse monoclonal antibodies (mAb) specific for chicken leukocyte markers (Southern Biotech, Birmingham, AL). All cell suspensions were dual labeled with either 1) chicken pan-leukocyte marker CD45- specific mAb conjugated to spectral red fluorochrome (SPRD) together with phycoerythrin (PE)-conjugated mAb specific for the chicken monocyte/macrophage marker KUL01 (KUL01-PE), or 2) mAb specific for the chicken B cell marker Bu-1 conjugated to PE (Bu1-PE) together with the mAb specific for the chicken T cell marker CD3 (CD3-SPRD). The isotype of all mouse mAb used was IgG1. The leukocyte-specific antibodies, along with PE- and SPRD-conjugated isotype controls (Southern Biotech) to identify nonspecific binding of mAb, were diluted 1:100 in PBS+ (PBS, 1% bovine serum albumin, and 0.1% sodium azide). Two-color cell population analysis was conducted as described in Erf and Ramachandran (2016) using a BD C6 Accuri flow cytometer (Becton Dickinson, San Jose, CA) and FlowJo software v.10.5 (Ashland, OR). The heterophil population was identified based on size (FSC) and granularity (SSC) characteristics of CD45+ leukocytes (Seliger et al., 2012). All data were expressed as the percentage of stained cells in the total pulp cell suspension (% pulp cells).
Reactive-Oxygen-Species Generation Assay
Reactive-oxygen-species generation was determined for each pulp cell suspension by kinetic fluorescence assay using 2′,7′-dichlorofluorescin-diacetate (DCFDA, Sigma, St. Louis, MO) (Rath et al., 1998). Briefly, 100 μL of each cell suspension was added to 100 μL of room-temperature PBS in triplicate wells of a 96-well microtiter plate, and incubated for 30 min at 37°C. After incubation, 20 μL of DCF-DA solution (23 μg/mL) was added to each well. The plate was then transferred to a fluorescence microplate reader (Synergy HTX, BioTek, Winooski, VT) set at 37°C with an excitation wavelength of 485 nm and an emission wavelength of 530 nm. Generation of fluorescence from the samples was analyzed via kinetic read for 1.5 h at 10-min intervals using Gen5 software (BioTek). To ensure multiple readings for each sample within the fluorescence detection range, pulp cell suspensions prepared from 0-, 6-, and 24-h samples were used undiluted or diluted 1:4 or 1:2 with PBS, respectively. Appropriate controls for autofluorescence and background fluorescence were included in each assay. A line of best fit describing the relationship between fluorescence (a.u.) and time was calculated for each sample and the relative amount of ROS generation reported as the slope of the best fit equation.
Blood Cell Profile Analysis
Half of each blood sample (1 mL) collected from all 24 birds at each time point was subjected to automated hematology analysis (Cell-Dyn; Abbott Diagnostics, Abbott Park, IL). Data acquired included proportions (% of leukocytes) and concentrations (103/μL) of heterophils, monocytes, lymphocytes, basophils, and eosinophils, concentrations of total leukocytes (103/μL), thrombocytes (103/μL), and red blood cells (RBC; 106/μL), as well as hemoglobin (g/dL) and hematocrit (g/dL).
Superoxide Dismutase Activity
Superoxide dismutase (SOD) activity in both GF pulps and plasma samples was determined using a SOD assay kit (Cayman Chemical, Ann Arbor, MI) following the manufacturer’s protocol with modifications on the preparation of homogenates from GF pulps. Briefly, to prepare pulp homogenates from 1 GF per bird and time point, the entire pulp was removed from the feather sheath, weighed, and placed in a 1.5-mL microcentrifuge tube containing a 10x volume (10 μL/mg pulp) of ice-cold 20 mmol/L HEPES buffer (pH 7.2, 1 mmol/L EGTA, 210 mmol/L mannitol, and 70 mmol/L sucrose) and an equal weight (1 mg beads/ mg pulp) of 0.5-mm zirconium oxide beads (Next Advance, Inc., Troy, NY). Tubes were then placed in a Bullet Blender (Next Advance) to homogenize pulps at Speed 12 for 2 cycles (5 min/cycle) at 5°C. Homogenates were then centrifuged at 10,000 x g for 15 min. Supernatant was collected and diluted 1:8 with HEPES buffer. Seven standards (ranging from final SOD activity levels of 0–0.05 U/mL) were made fresh in accordance with Cayman Chemical’s protocol and kept on ice until the SOD assay was performed. All reagents provided in the kit (assay buffer, sample buffer, radical detector, SOD standard) were added to the assay plate in accordance with the kit protocol along with 10 μL of diluted pulp homogenates. Finally, 20 μL of xanthine oxidase was added to each well to generate reactive oxygen species. The assay plate was then incubated on a plate shaker for 30 min at room temperature. During this time, xanthine oxidase reacts with superoxide anions and tetrazolium salt (radical detector) to produce formazan dye (yellow in color). After the 30-min incubation, dye absorbance at 450 nm was measured in a microplate reader (Synergy, BioTek) and Gen5 software (BioTek).
Plasma SOD activity was measured in plasma samples diluted 1:25 (predetermined optimal dilution) in sample buffer following the same assay procedures described above. For both pulp and plasma samples, SOD activity was calculated in accordance with the manufacturer’s protocol and expressed as U/mL.
Total RNA Isolation and Quantification, and cDNA Synthesis From Flash-frozen GF Pulp Tissue
Growing feather samples that were flash-frozen in liquid nitrogen and stored at −80°C were equilibrated to cold room temperature (5°C). For each bird and time point, pulps were then extracted from the feather sheaths, and dropped into 1.5-mL Safe-Lock microcentrifuge tubes (Eppendorf, Hauppauge, NY) containing 200 μL of TriReagent (Zymo Research Corp., Irvine, CA) and 20 ± 3 mg of 0.5-mm zirconium oxide beads (Next Advance). The tubes were placed in the Bullet Blender (Next Advance) set to homogenize the tissue at Speed 12 for 2 cycles (5 min/cycle) at 5°C. Tubes were centrifuged at 12,000 x g for 5 min and supernatant fluid pipetted into a fresh 1.5-mL microcentrifuge tube containing ice-cold ethanol (100%). Total RNA was isolated from the homogenates using the Directzol kit (Zymo Research), following the manufacturer’s protocol for tissue samples with in-column DNase digestion. Total RNA quality and concentration were determined using NanoDrop (Thermo Fisher Scientific, Waltham, MA), and all available total RNA (0.8–5 μg) was transcribed to cDNA using the High-Capacity cDNA kit with the MultiScribe Reverse Transcriptase, following the manufacturer’s protocol (Thermo Fisher Scientific). All cDNA samples were diluted to a working concentration of 10 ng/μL with nuclease-free water and stored at −20°C until used for relative gene expression analyses.
Relative Gene Expression Analyses
Target gene primers and probes used in this study are listed in Table 1. Duplicate 10-μL reactions were prepared in accordance with the TaqMan Universal Master Mix with UNG protocol (Thermo Fisher Scientific), using final concentrations of 10 ng cDNA, 0.1 μmol/L probe, and 0.1 μmol/L of each primer. Quantitative real-time PCR was performed in a 7500 Fast Real-Time PCR System (Thermo Fisher Scientific) with the following temperature profile: hold stages of 50°C for 2 min and 95°C for 10 min, followed by 40 cycles of denaturing (95°C for 15 s) and annealing (60°C for 1 min). Given that all primer-probe assays had similar PCR efficiencies (1.52 ± 0.064), the cycler was programmed to analyze expression data using the comparative CT (ΔΔCT) method (Schmittgen and Livak, 2008), with 28S as the reference gene (Borowska et al., 2016) and a pool of cDNA prepared from noninjected feather pulps as the calibrator sample. The expression profile of each gene target in a treatment sample is presented as a fold change in expression relative to the calibrator sample.
Statistical Analyses
The LPS was injected into GF of individual birds, and as such, bird was considered the experimental unit. For all measurements, data were analyzed by a 3-way repeated measures analysis of variance (RM ANOVA) using JMP 12 software (SAS Institute Inc., Cary, NC) to determine effects of time, diet, and gender, and time by diet, time by gender, diet by gender, and time by diet by gender interactions, followed by Tukey’s multiple means comparisons as appropriate. In all cases, statistical significance was considered at P ≤ 0.05.
RESULTS
Effects of Intradermal Pulp Injection of Lipopolysaccharide on Inflammatory Activities at the Site of Injection and in the Peripheral Blood Circulation
For all aspects examined, 3-way repeated measures ANOVA revealed no 2- or 3-way interactions (P > 0.05) and no gender or diet effects (P > 0.05).
Hence, data across gender and diet are reported in the current article with focus on the main effect of time and comparisons of data before (0 h) and at 6 and 24 h after intradermal injection of PBS or LPS into the pulp of GF (n = 8 or n = 24 per time point, respectively).
Leukocyte Population Profiles in the Pulp of Growing Feathers Before and After Injection of PBS or LPS
Vehicle injection (10 μL endotoxin-free PBS) into GF of 8 broilers resulted in a slight elevation (P = 0.089) of heterophils at 6 h (i.e., 2.32, 4.45, and 2.48 ± 0.71% at 0, 6, and 24 h, respectively), but did not affect macrophage, and T- and B-lymphocyte levels (Table 2).
After GF injection of LPS, levels of heterophils (% pulp cells) increased from 1.72 ± 0.98% (main time effect mean ± SEM) before injection (0 h) to highest levels (25.1 ± 0.98%) at 6 h after LPS injection (P < 0.0001), and remained elevated at 24 h, although at lower levels (17.8 ± 0.98%) than at the 6 h time point (P = 0.0002) (Table 2, Figure 1). Macrophage levels increased (P = 0.011) from 1.98 ± 0.27% at 0 h to 3.12 ± 0.27% at 6 h, and again (P < 0.0001) to 8.43 ± 0.27% at 24 h (Figure 1A). Lymphocyte levels were higher before than after LPS injection (P = 0.003), with levels dropping from 2.15 to 1.53, and 1.23 ± 0.14% at 6 (P = 0.0235) and 24 h (P = 0.0032), respectively (Figure 1). This drop in pulp lymphocytes after LPS injection was due to a proportional drop in both T- and B-cells (Table 2).
Concentration and Proportions of Cells and Components in the Peripheral Blood Circulation
Intradermal injection of LPS into the pulp of GF affected the concentration (103/μL) of various blood leukocytes (Table 3). Similar to observations in the pulp, heterophil concentrations increased (P < 0.0001) from 8.36 ± 1.34 x 103/μL at 0 h to highest levels (29.01 ± 1.34 x 103/μL) at 6 h after LPS injection, but then returned to preinjection levels (P = 0.8654) by 24 h (9.33 ± 1.34 x 103/μL). Monocyte concentrations increased (P = 0.0017) from 0.89 ± 0.17 x 103 /μL at 0 h to highest levels (1.85 ± 0.17 x 103/μL) at 6 h after LPS injection and returned to preinjection levels (P = 0.6622) by 24 h (0.68 ± 0.17 x 103/μL). Blood lymphocyte concentrations decreased (P =0.0114) from 8.58 ± 0.72 x 103 /μL at 0 h to 5.35 ± 0.72 x 103/μL 6h after LPS injection, but then increased again (11.44 ± 0.72 x 103/μL) at 24 h to levels higher (P = 0.0266) than before LPS injection. The concentrations of basophils changed in response to LPS injection (P = 0.0125), dropping from 0.99 ± 0.14 x 103/μL at 0 h to 0.56 ± 0.14 x 103/μL at 6 h (P = 0.0872), and returning to preinjection levels (P = 0.6190) by 24 h (1.17 ± 0.14 x 103/μL). Concentrations of eosinophils did not change in response to LPS injection into GF pulp (P = 0.8654) (Table 3).
Examination of the proportions among blood leukocyte populations (Figure 1C) revealed similar trends in the percentages of heterophils, lymphocytes, basophils, and eosinophils than those described for their respective concentrations (Table 3). However, unlike the concentration of monocytes, the percentage of monocytes was not affected by LPS administration (Table 3).
Total white blood cell concentrations followed the same overall trends observed for the heterophil and monocyte populations with concentrations increasing (P < 0.0001) from 18.6 ± 1.68 x 103/μL at 0 h to 36.5 ± 1.68 x 103/μL at 6 h after LPS injection, and returning to preinjection levels (P = 0.2763) by 24 h (22.3 ± 1.68 x 103/μL). Thrombocyte concentrations were similar (P = 0.6494) at 0 h (22.9 ± 1.49 x 103/μL) and 6 h (24.8 ± 1.49 x 103/μL) after LPS injection but increased (P = 0.0023) to 31.0 ± 1.49 x 103/μL by 24 h. Red blood cell concentrations decreased (P = 0.0049) from 2.44 ± 0.07 x 106/μL at 0 h to 2.19 ± 0.07 x 106/μL at 6 h after LPS injection and returned to preinjection levels (P = 0.9796) by 24 h (2.43 ± 0.07 x 106/μL) (Table 3). Hemoglobin and hematocrit concentrations followed the same trend as RBC concentrations (Table 3).
Reactive-Oxygen-Species Generation and Superoxide Dismutase Activity
Intradermal injection of LPS into the pulp of GF resulted in heightened (P < 0.0001) ROS generation in pulp cell suspensions at 6 h compared with 0 h (3,129.1 vs. 254.4 ± 264.4 U/min; Figure 2). By 24 h, ROS generation had returned to preinjection levels (P = 0.9142). Superoxide dismutase activity in pulp homogenates was slightly higher (P = 0.042) at 6 h after than before LPS injection (6.38 vs. 5.43 ± 0.34 U/mL) and dropped (P = 0.3363) at 24 h to levels intermediate to those at 0 and 6 h. In the plasma, SOD activity (U/ mL) was elevated at 24 h (13.75 ± 0.65) compared with samples collected before (9.08 ± 0.65; P < 0.0001) and at 6 h (10.04 ± 0.65; = P 0.0011) after LPS injection into the pulp (Figure 3).
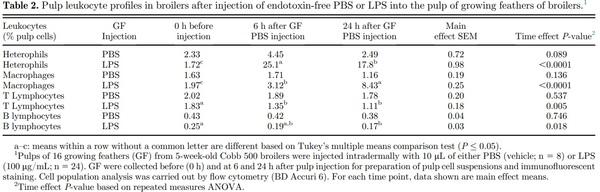
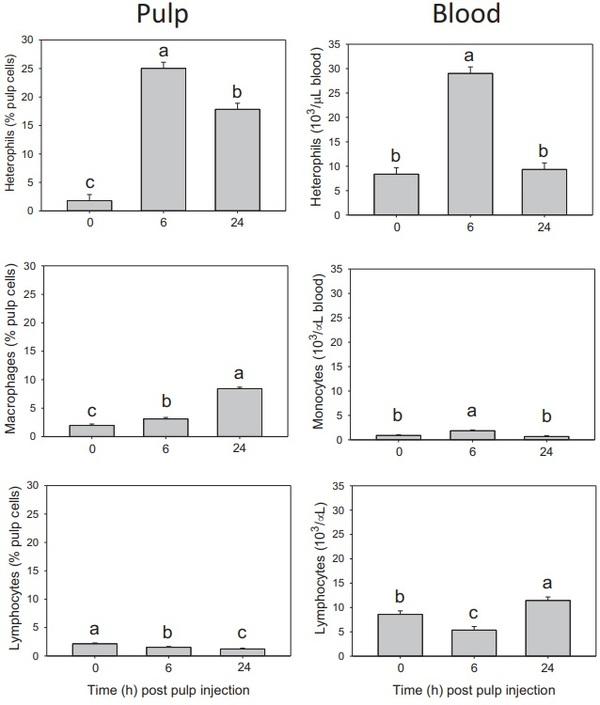
Figure 1. Leukocyte profiles in pulp of growing feathers (GF) and blood at 0, 6, and 24 h after intradermal pulp injection of lipopolysaccharide (LPS). Pulps of 16 GF from each of 24 5-week-old Cobb 500 broilers (12 males and 12 females) were injected intradermally with 10 μL of LPS (100 μg/mL; 1 μg/GF). A) Two GF from each chicken, collected before (0 h) and at 6 and 24 h after pulp injection of LPS were used to prepare pulp cell suspensions. Pulp cell suspensions were stained with a panel of fluorescence-conjugated mouse monoclonal antibodies (Southern Biotech) to identify chicken macrophages (KUL-01) and lymphocytes (CD3 and Bu-1). Cell populations were analyzed by flow cytometry (BD Accuri 6) and FlowJo software (v. 10.5.3). Heterophil populations were gated based on size (FSC) and granularity (SSC) characteristics of leukocytes (CD45+). B) Concentration (103 cells/μL) and C) proportions (%) of blood leukocytes were determined using an automated hematology analyzer (Cell-Dyn, Abbott Core Laboratory). All data shown are time main-effect means ± SEM (n = 24). For each cell type, means without a common letter are different (P ≤ 0.05).
Relative Expression of Inflammation-Related Genes in LPS-injected Pulps Injection of LPS into the pulp of GF resulted in increased RNA expression of IL1-β (P < 0.0001), IL-6 (P = 0.011), IL-8 (P < 0.0001), IL10 (P < 0.0001), and cathelicidin B1 (P = 0.0059) genes, but not of LPS-induced TNF-α factor (LITAF), TNF-α, SOD1, and SOD2 (Figure 3). Of the upregulated target genes examined, IL-1β, IL-8, and IL-10 reached highest (P < 0.0001) expression levels of approximately 100-, 40-, and 100-fold, respectively, at 6 h, and remained elevated (P < 0.001) at 24 h. Cathelicidin B1 expression level was elevated only at 24 h (P = 0.0065), increasing approximately 5-fold over preinjection level. A 5-fold increase from the preinjection level was also observed for IL-6 at 6 and 24 h after LPS injection. Levels of LITAF, TNF-α, SOD1, and SOD2 expression were found to be lower (P < 0.0001) after than before LPS administration (Figure 3).
DISCUSSION
Inflammatory responses to microbes and other stimulants occur in complex tissues where containment and elimination of the infection depends on local innate immune system activities designed to recruit blood leukocytes and proteins to the affected tissue. The present study is the first to describe the early phase (6 and 24 h) of the acute inflammatory response in commercial broilers by simultaneously monitoring activities initiated by LPS at the site of injection (i.e., in the GF pulp) and in the peripheral blood circulation in the same individual. Using this dual-window approach, important new knowledge regarding temporal, quantitative, and qualitative alterations observed in the blood and local injection site were revealed that warrant consideration when evaluating the inflammatory response in chickens.
Local (Pulp) and Systemic (Blood) Cell Population Changes in Response to Pulp Injection of LPS
As expected, heterophils were the first and most abundant cell type recruited to the site of LPS injection followed by infiltration of monocyte/macrophages, reaching maximal frequency at 6 h and 24 h, respectively. On the other hand, lymphocytes were not recruited to the site of LPS injection during this time. In fact, the frequency of both T- and B-lymphocytes dropped proportionately at 6 h and 24 h after LPS injection. The reduction in lymphocyte frequency after LPS injection is likely a reflection of the increased presence of heterophils and macrophages in the pulp cell suspensions rather than lymphocyte emigration or death.
Heterophil and monocyte concentrations also were increased in the blood at 6 h after GF pulp injection of LPS. However, although in the pulp heterophil frequency remained elevated and that of macrophages increased further, concentrations of heterophil and monocytes in the blood returned to preinjection levels at 24 h. The increase in blood heterophil concentrations at 6 h was accompanied by a drop in the concentrations of lymphocytes and basophils. Although basophil concentrations returned to preinjection levels, those of lymphocytes reached highest concentrations at 24 h. Eosinophil concentrations did not change after LPS injection. Most of the alterations in blood leukocyte concentrations after LPS injection into GF were also reflected in the proportions (%) among leukocyte populations, with the exception of no changes in proportions of monocytes and a return of the lymphocyte proportions to preinjection levels at 24 h. Overall, from these observations, it may be concluded that determination of blood leukocyte concentrations and proportions are indicative of an LPS-induced inflammatory responses taking place in a tissue, although they do not fully reflect leukocyte profiles at the site of LPS injection. In addition, leukocyte concentration data more accurately reflect alterations in blood leukocyte profiles than data on the proportions among various leukocyte populations.
Similar alterations in blood leukocyte levels have been described by others after LPS was administered by i.d., intravenous (i.v.), intramuscular (i.m.), intraabdominal (i.a.), or subcutaneous (s.c.) injection in chickens (Shini et al., 2008; Bowen et al., 2009; De Boever et al., 2009; Byrne, 2016; Lieboldt et al., 2017). In addition, studies examining histology sections prepared from LPSinjected tissues described similar heterophil and monocyte/macrophage infiltration profiles. For most of these studies, the dose of LPS administered was much greater than in the present study with doses exceeding 1 mg/kg in several cases (Smith et al., 1975; Qureshi et al., 1986; Qureshi, 2003; Shini et al., 2008; Bowen et al., 2009; Chang et al., 2020). Chickens, especially broilers, are known for their high level of tolerance to LPS (Leshchinsky and Klasing, 2001). Hence, it is surprising that the low dose of LPS administered by GF injection (i.e., 16 GF injected with 1 mg LPS each; 7–8 mg/kg) not only resulted in local inflammatory activity, but also in a clearly measurable response in the blood, with the classic alterations in leukocyte profiles ascribed to the inflammatory response across various species (Lieboldt et al., 2017; More Bayona et al., 2017; Abbas et al., 2018).
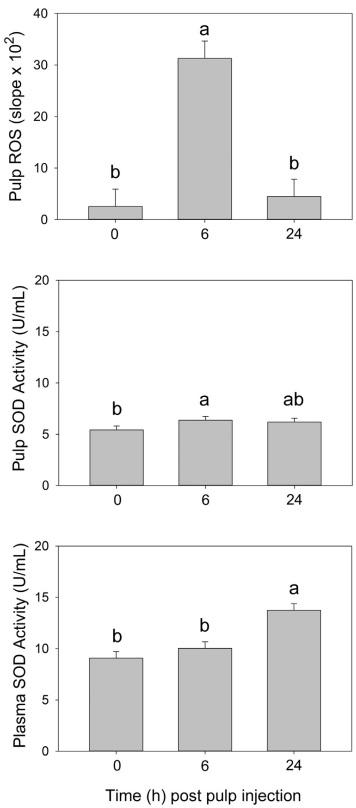
Figure 2. Reactive-oxygen-species (ROS) generation and activity of superoxide dismutase (SOD) in pulp tissue and plasma at 0, 6, and 24 h after intradermal injection of lipopolysaccharide (LPS) into pulps of growing feathers (GF). Pulps of 16 GF from each of 24 5-week-old Cobb 500 broilers (12 males and 12 females) were injected with 10 μL of LPS (100 μg/mL; 1 μg/GF). Two GF from each chicken, collected before (0 h) and at 6 and 24 h after pulp injection of LPS were used to prepare pulp cell suspensions. Pulp cell suspensions were used to determine ROS generation by kinetic fluorometric microplate assay. In addition, 1 GF and blood collected from each broiler at each time point were used to prepare pulp homogenates and plasma for assessment of SOD activity by colorogenic (450 nm) microplate assay (Cayman Chemical). Data shown are time main-effect means ± SEM (n = 24). For each cell type, means without a common letter are different (P ≤ 0.05).
The observed increase in blood heterophils at 6 h is in line with reports of LPS- and inflammation-stimulated release of heterophils from the bone marrow and influx into the blood (Shini et al., 2008; More Bayona et al., 2017). The drop in blood lymphocyte concentrations within hours of LPS administration also was observed by others (Shini et al., 2008; Bowen et al., 2009) in egg-type chickens after i.v. injection of high doses (> 1 mg/kg BW). Further examination revealed LPSinduced lymphocyte degeneration and destruction rather than redistribution to the lung, liver, or spleen, or firm vascular adhesion (Shini et al., 2008; Bowen et al., 2009).
Similar changes in the proportions of heterophils to lymphocytes also are associated with physiological stress (Davison and Flack, 1981; Gross and Siegel, 1983). It should be noted that the effects of the vehicle injection into GF on blood leukocyte profiles were not examined in this study. Therefore, it is not known whether part of the observed alterations was due to handling associated and sample collection-associated stress. Considering that PBS injection into GF 1) was repeatedly shown not to affect blood leukocyte profiles (Byrne, 2016; Erf et al., 2017), 2) the 24-h blood samples had baseline proportions of heterophils and lymphocytes, and 3) PBS injection of GF did not alter leukocyte proportions beyond a low, statistically nonsignificant, elevation in heterophils; we are confident that the observed alterations in blood leukocytes profiles are due to LPS administration.
In addition to alterations in blood leukocyte levels, concentrations of thrombocytes increased at 24 h after LPS injection into GF pulp and those of RBC, hematocrit and hemoglobin dropped at the 6 h time point. The increase in circulating thrombocytes during the inflammatory response induced by injection of LPS in the GF pulp may be due to their role in vascular tissue repair (Ferdous and Scott, 2014). In addition, LPS has been shown to directly activate thrombocytes to produce proinflammatory cytokines and chemokines, like those produced by macrophages (Ferdous and Scott, 2015; Ferdous et al., 2017). Hence, thrombocytes may contribute to the LPS-induced inflammatory response. By contrast, the drop in RBC levels, hematocrit and hemoglobin, may be due to direct cytotoxicity of LPS and factors produced during the inflammatory response, as reported by Bateman et al. (2017) for mammalian RBC. More research is needed to gain further insights into this phenomenon in chickens.
Local (Pulp) Inflammation Related Activities in Response to Pulp Injection of LPS
One of the first activities of LPS-activated phagocytes, such as heterophils and macrophages, is the respiratory burst that results in increased generation of ROS with potent antimicrobial effects (Farnell et al., 2003). Here, ROS generation was measured in the same pulp cell suspensions used to determine leukocyte infiltration profiles. Considering that ROS generation and heterophil levels in GF pulp showed a congruent course, ROS generation appears to be primarily due to heterophil activation. As ROS can cause tissue injury, antioxidant enzymes, such as SOD are also activated during the inflammatory response to offset the harmful effects of ROS, specifically superoxide anions (Surai, 2015; Perez et al., 2017). Examination of SOD activity levels in homogenates prepared from LPS-injected GF pulps revealed heightened SOD activity compared with the preinjection controls. It is likely that both heterophils and macrophages contributed to the local SOD activity (Surai, 2015; Perez et al., 2017; Ighodaro and Akinloye, 2018). It is interesting that SOD activity measured in the plasma of the same animals at the same time points was elevated at 24 h. Additional studies are needed to identify the source of the extracellular SOD activity observed in plasma during the LPS-induced inflammatory response in broilers.
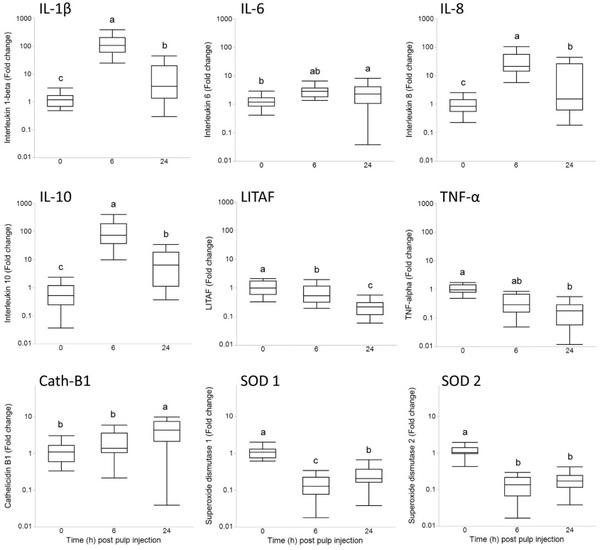
Figure 3. Quantile box plots of relative gene expression in pulp tissue at 0, 6, and 24 h after intradermal injection of lipopolysaccharide (LPS) into pulps of growing feathers (GF). Pulps of 16 GF from each of 24 5-week-old Cobb 500 broilers (12 males and 12 females) were injected with 10 μL of LPS (100 μg/mL; 1 μg/GF). Total RNA was isolated from the pulp of one flash-frozen GF collected from each chicken before (0 h) and at 6 and 24 h after LPS injection and used for quantitative RT-PCR gene expression analyses by the comparative CT (ΔΔCT) method on the 7500 Fast Real-Time PCR System (Applied Biotechnologies). Data shown are time main-effect means of fold change in expression ± SEM (n = 24). For each gene target, means without a common letter are different (P ≤ 0.05), based on statistics performed on ΔCT data. The hollow spaces in the box represent the quartiles separated by a horizontal line at the median.
Targeted gene expression analysis by quantitative RT-PCR revealed that intradermal injection of GF pulps with LPS resulted in upregulation of proinflammatory cytokines IL-1β and IL-6, as well as chemokine IL-8, whereby expression of IL-1β and IL-8 was substantially greater than that of IL-6 and was already elevated at 6 h. Cytokine IL-10, known to have anti-inflammatory activity (Wu et al., 2016), also was upregulated in a similar pattern to proinflammatory cytokine IL-1β, presumably to control the local inflammatory response. These expression profiles are in line with reports by others based on LPS activation of isolated heterophils and macrophages, as well as the spleen and other tissues after i.v., s.c., and i.a. LPS administration (Kaiser et al., 2012; Kaspers and Kaiser, 2014; Byrne, 2016; Perez et al., 2017; Oh et al., 2019; Chang et al., 2020). Surprisingly, expression of LITAF, a factor involved in transcriptional regulation of the proinflammatory cytokine TNF-α (Hong et al., 2006), as well as that of the recently characterized TNF-α gene in chickens (Rohde et al., 2018), were not upregulated during the inflammatory responses initiated by LPS in GF pulp. Rohde et al. (2018) reported expression of TNF-a within hours after LPS activation of chicken macrophages (spleen-, bone marrow-, and monocyte-derived) and lymphocytes, especially CD41 T cells. Analysis of the spleen and liver tissue after s.c. administration of 10 μg LPS/kg in chickens, revealed increased expression of TNF-α in the spleen, but not in the liver, 3 h after LPS administration, whereas IL-6 expression was upregulated in both tissues (Rohde et al., 2018). These and our observations suggest that TNF-α expression is regulated by LPS in a different manner than the other proinflammatory cytokines. Moreover, it is conceivable that the recently recruited monocytes, which appeared later than heterophils, in LPS-injected GF may be in the process of differentiating into macrophages and were not at a functional stage to initiate LITAF and TNF-α gene transcription. Another reason for undetectable TNF-α expression in this early phase of the LPS-induced inflammatory response in GF may be the lack of lymphocyte participation. The GF cutaneous test system offers excellent opportunity to examine TNF-α' s expression and role in the avian inflammatory response.
Expression of phagocyte function–related genes such as cathelicidin B1, an antimicrobial peptide, was also upregulated 24 h after LPS injection into GF pulp. Similar observations were reported by Perez et al. (2017) in monocytes isolated after LPS administration in chickens. Although SOD enzyme activity was elevated in LPS-injected pulps, expression of SOD1 and SOD2 genes were not upregulated after LPS injection. Thus, it is likely that an inactive form of SOD protein stored in heterophil granules and monocyte lysosomes was activated by LPS-induced mechanisms (Manni et al., 2011).
In summary, we successfully adapted the GF tissue test system for use with broiler GF, providing an important new tool to monitor and assess innate immune system capabilities in these young, fast-growing animals. The dual window approach used to monitor the responses to tissue injection of LPS, both at the local injection site and in the peripheral blood circulation in the same individuals, is a more informative approach than sole examination of inflammatory activities in the blood. New insights into temporal, qualitative, and quantitative aspects of the immunostimulatory effects of LPS at the site of injection were revealed. Although classic leukocyte infiltration profiles were observed, new questions emerged regarding functional differentiation and activities of recruited cells, that can be further dissected using the GF “in vivo test-tube” system. Hence, this study provides the basis for more defined research into the inflammatory response to LPS and other pathogen and damage-associated molecular patterns in chickens. New knowledge generated from further dissection and comprehensive analysis of innate immune responses may find direct application in identification of individuals with robust, balanced innate defenses and provide a platform for studying the immunomodulatory effects of exogenous treatments (e.g., nutrients, probiotics, immunostimulators, etc.).