1. Introduction
The scope of this review is limited to agricultural concrete and metallic structures exposed to a corrosive environment. The content of the review paper includes the following topics and subtopics: agricultural concrete and metallic structures (impact of poultry, cattle, and swine manure), specific impact of corrosion on steel structures, corrosion, and degradation of metallic structures (corrosion in the farm environment, activity of fungi and bacteria, durability of concrete and acid-induced corrosion, mechanisms of acid corrosion) and corrosion control measures. The main problem under investigation was the mechanisms that contribute to the degradation of agricultural buildings that are in contact with agricultural effluents, animal manure, and natural elements. Additionally, the most effective mechanisms to mitigate corrosion were explored; a higher emphasis was placed on the design for durability, silica fumes, and fly ash ratios in concrete, sulfur concrete, and fiber-reinforced polymers.
Corrosion predicts the durability of structural materials used in farms and agricultural environments, which are characterized by bio-waste, agro-food effluents, silage juices, manure, and molasses, that originate from agricultural processes [1]. Other factors that enhance the risk of corrosion include high concentrations of acids (originating from spilled meal-water mixtures), salts, chlorine-containing sterilizing chemicals, aggressive gases, hydrogen sulfide (H2S), dust, and temperature [2]. In addition, microbes—such as fungi, yeast, and bacteria—contribute to microbial induced corrosion [2]. The aim of this review article is to contribute new knowledge concerning the durability of concrete structures exposed to aggressive corrosion linked to acids, moisture, bacteria, and other corrosion-inducing agents and the role of high strength concrete, special concretes, stainless steel and galvanized steel in the mitigation of corrosion and the environmental degradation of materials.
2. Agricultural Concrete and Metallic Structures
De Belie and co-researchers [2] noted that steel reinforcements are commonly found in cow and pig precast concrete housing for pigs and cows, weaned (any weaned animal), and farrowing houses for pigs. Other common areas that are made of steel structures include downpipes, gutters, milking parlors, and partitions for cows and other domestic animals. Similarly, cast-iron and galvanized steel are commonly used to reinforce stalls for dairy cattle. In brief, metal reinforcements are integrated into different structures to improve the structural integrity of the concrete. The integration of steel into standard concrete structures exacerbates the risk of metal and concrete corrosion; this is because metals are highly susceptible to high humidity levels, temperature changes, and elevated concentrations of corrosive gases such as carbon dioxide (CO2) and hydrogen sulfide (H2S). However, metals react following exposure to chemicals, as noted in Table 1.
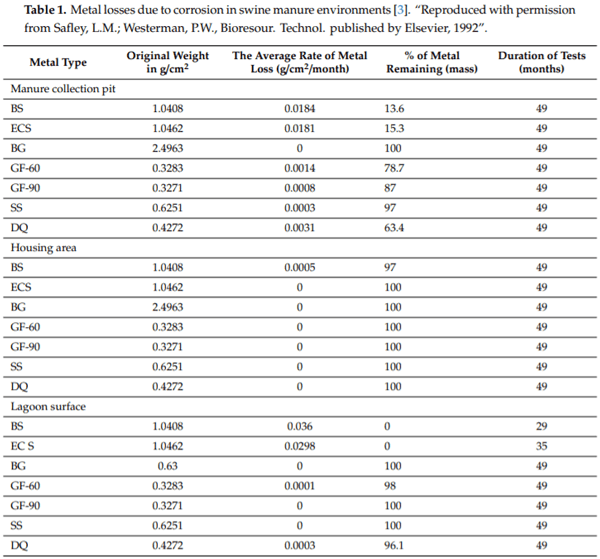
The impact of different corrosive agents in Table 1 shows that corrosive gases, which are commonly found in silage and feed residues, accelerate corrosion through the formation of carbonic acid and sulphuric acid (in the presence of sulfate ions), respectively. The action of sulphuric acid and other corrosive agents on concrete structures is outlined below.
Materials and chemicals that are responsible for corrosion in farm environments include pure water at a pH of 7. Water mainly originates from snow, spring water, morning dew, or rain [1,2]. Water is associated with the dissolution of concrete and leaching into the microstructure. In addition to water, inorganic acids—such as sulphuric acid, nitric acid, carbonic acid, and phosphoric acid—which originate from natural waters, chemical industry effluents have the potential to corrode the concrete structures. The extent of corrosion is influenced by the strength of the acid–acid dissociation constant (Ka) and pH. Diary areas and animal fodder silos, manure, and silage are among the lead sources of inorganic acids—such as oxalic, formic, tannic, lactic, and acetic acids—which dissolve into the concrete structures and mitigate the setting process [1,2]. The carbonic acid formed poses a risk to the concrete and steel structures in the farm environment due to the transition to higher acidity values. Acid attacks impact the durability of farm structures. Corrosion of concrete structures can also be induced by oxalic and humic acids from chemical factories, contaminated ballast, and soils. Humic acid impacts the strength of concrete and accelerates the corrosion process. Even though oxalic acid has no known adverse effect on concrete at mild concentrations, it can dissolve concrete at high concentrations. The impact of organic acids on metallic structures is similar to alkali chemicals.
Plants and animal oils and fats from the food industry loosen the concrete structure. The loosening process (separation of the concrete constituents) is characterized by the reaction of the fatty acids with calcium salts to form soft calcium soaps. In addition, organic and inorganic acids, ions such as magnesium and ammonia and sulfate found in industry effluents, artificial fertilizers, natural water, and effluent, increase the rate of concrete swelling, accelerate concrete dissolution and soften the concrete structures. Similar corrosive and dissolving action has also been observed in the acidic solutions with a pH of less than 6.5, CO, CO2, and H2CO3 and hydrogen sulfide gas originating from feed residues, and silage [1,2].
2.1. Impact of Poultry, Cattle Manure, and Swine Manure on Corrosion of Concrete and Metals
Poultry and swine manure have different corrosive effects on farm structures. The observation is based on Safley and Westerman research, which explored the impact of manure over a period of 49 months [3]. The research purposed to determine whether the risk of corrosion was ubiquitous following exposure to cattle, swine, and poultry manure. The experiments were conducted using different metal specimens namely drawing quality galvanized steel (DQ), stainless steel (SS), Galfan 90 (GF-90), Galfan 60 (GF-60), batch galvanized (BG), cold-rolled steel covered with epoxy paint (ECS), and unpainted cold-rolled steel (BS), which were exposed to different forms of manure in the anaerobic lagoon (submerged and suspended), animal housing, and manure collection pit.
The data depicted in Tables 2–4 show that cold-rolled steel was most susceptible to corrosion in the housing section. Similarly, cold-rolled still was susceptible to corrosion in the lagoon section. In contrast, drawing quality steel had the highest rate of corrosion in the manure collection point, followed by stainless steel and Galfan 90 [3]. The inconsistencies in the rate of corrosion among different metals exposed to different environments could be attributed to the variations in the corrosive species such as chloride ions, chemical oxygen demand, total dissolved solids, chloride ions, and potassium, sodium, magnesium, and calcium ions. The observations made by Safley and Westerman concerning the variable chemical composition of different types of manure are in line with findings made by Belie and co-researchers using the Kjeldahl method [4]. Another notable observation was that there was a considerable variation in the composition of poultry, swine, and cattle/dairy manure between 1985 and 1990. The changes could be linked to the composition of the animal feeds, water, or environmental factors.
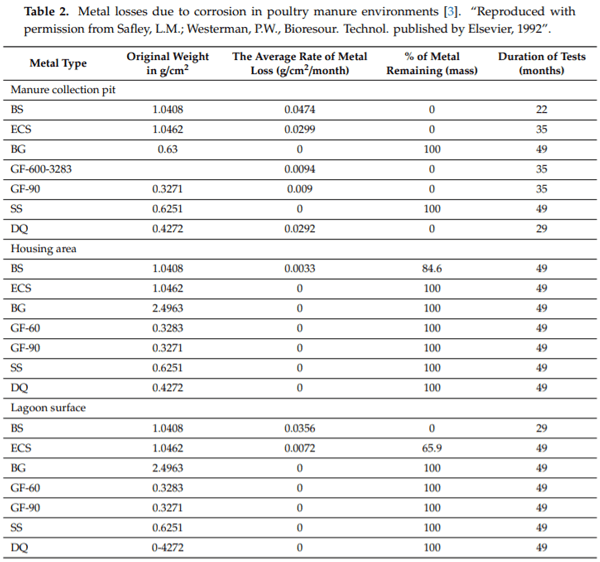
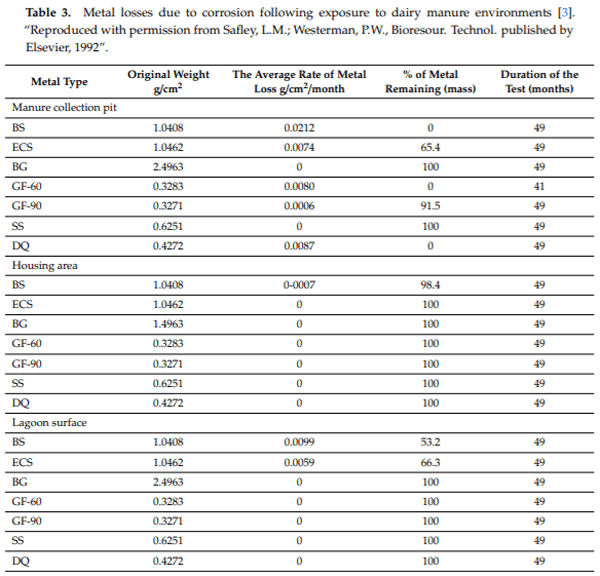
Based on the 49-week experiments concerning the extent of metal loss after exposure to manure lagoon, manure collection pit and feeding area, galvanized steel, metal plate, and stainless steel offered the optimal protection against corrosion in each of the three forms of manure. Galfan 60 and Galfan 90 had near equal levels of corrosion resistance after prolonged exposure to manure [3]. The corrosion of metallic structures, which are in contact with manure and animal waste, have significant economic implications. Schouten and Gellings reported a depreciation rate of about 50% over a three year period [5]. In addition, the corrosion-induced depreciation of the metal structures was influenced by the type of farming—arable or dairy farming. The risk of corrosion was most pronounced in agricultural structures associated with arable farming (63% of the corrosion costs). However, the risk of corrosion was mitigated by coating, galvanization, and passivation.
An investigation of the impact of the chicken coop on the cement microstructure by Strokova and co-researchers made the following observations. There were variations in the degradation of the cement structure depending on the area of operation (poultry excrement section, floor with wood chips, and hay and aerial section). The section of the concrete that was exposed to chicken coop was highly eroded compared to other sections due to microbiological corrosion. Chicken waste contains significant quantities of H2S and NH3 and corrosive agents, which cause corrosion through the mechanisms discussed in Table 1.
In addition to the corrosive substances found in chicken coop, concrete structures which come into contact with chicken coop corrode due to regular cleaning with disinfectants which are made of corrosion inducing agents such as hydrogen peroxide (H2O2), formalin, peroxyacetic acid, soda ash, slaked lime, iodine monochloride, glutaric aldehyde, neutral calcium hypochlorite, lime chloride, and caustic soda [6]. The cumulative effect was a 10% loss in the strength of the concrete and irreparable damage to the microstructure, as illustrated in the SEM images in Figure 1; the damage extended to both the upper and the lower layers. Figure 1a–h illustrate virgin structures and the microscopic changes defined by agglomerates from the products of hydration and the formation of crystals of calcite and final disintegration.
2.2. Specific Impact of Corrosive Agents on Steel Reinforced Concrete Structures
Metals are highly susceptible to corrosion following exposure to acids, alkalis, chloride, and ammonium containing solutions. The severity of the corrosive effect is influenced by the presence of H+ and OH- and the composition of the metallic structure. The incorporation of Cr-Ni into traditional steel increases the level of resistant to organic acid attacks [2]. Therefore, agricultural concrete structures reinforced by stainless steel, chromium steel, and chromium-nickel steels have a lower risk of structural failure compared to plain steel, aluminum, or zinc. On the downside, higher durability is o set by the costs associated with the treatment of the metallic structures. The impact of acetic acid on iron, steel, and zinc structures is considered in the next section.
The impact of acetic acid on steel and iron is dependent on concentration and temperature. On average, the material corrodes by about 0–79, 1–2.3, 1.34 mm/year in a concentration of 15–33% acetic acid, respectively [2,7]. The corrosion of chromium steel by acetic acid follows a similar approach as the corrosion of steel and iron. However, the risk of corrosion is considerably lower in chromium steel, given that the materials corrode by less than 0.11 mm/y in 10% acetic acid [2,7]. Austenitic chromium and nickel steel materials are resistant to acetic acid corrosion. The corrosion of chromium steel by acetic acid follows a similar approach as the corrosion of steel and iron. However, the risk of corrosion is considerably lower in Chromium steel, given that the materials corrode by less than 0.11 mm/y in 10% acetic acid [2,7]. Zinc structures are severely corroded, even in low concentrations of acetic acids 0.01 and 0.1% acetic acid [2,7]. However, the acid attack only occurs in the presence of oxidizing agents. Therefore, the elimination of oxidizing agents should diminish the risk of corrosion. Similar to other metals, the risk of corrosion is most pronounced at higher acid concentrations.
Iron and steel show some form of resistance to chloride solutions consisting of alkaline earth metals. The observation only applies if chlorides are present as solutions with alkali earth metals. However, the level of protection against corrosion is not absolute. Chromium steel is entirely resistant to corrosion induced by alkaline earth metals-chloride solutions. Chromium and nickel steel exhibit resistance against chlorides and chlorinated water. Zinc metal and structures exhibit weakened resistance to attack by acidic chlorides and chlorinated water. However, the risk of corrosion is substantially diminished in basic chloride solutions due to the formation of a protective layer. Aluminum structures have no resistance to chloride-containing solutions. However, the risk of attack is mitigated by the incorporation of inhibitors such as tolytriazole or sodium silicate [2,7]. Pitting corrosion has been observed following the exposure of aluminum to 10% calcium chloride solutions.
Steel structures are also corroded when exposed to gases containing ammonia, such as ammonia hydroxide. Therefore, the risk of corrosion could be partially mitigated by regulating the temperatures. Chromium-nickel steel structures are resistant to corrosion induced by ammonia containing solutions. Zinc structures are resistant to ammonia gas. However, they are susceptible to corrosion induced by ammonium hydroxide. The latter solution has been proven to lead to a rate of corrosion of about 0.14–0.37 mm/year in 3–55% ammonium hydroxide solutions, even at a neutral pH [2,7]. The risk of corrosion by ammonia and ammonium solutions is dependent on temperature— a limited amount of corrosion has been reported following the exposure of zinc to ammonia at higher temperature. However, the risk of elevated temperature does not apply in farm environments [2,7]. Higher temperatures apply in industrial settings. However, copper-containing Al alloys are an exception.
Iron and steel structures are susceptible to corrosion by ammonium salts—at a rate of 0.27 mm/yr in about 50 g/L of ammonium chloride [2,7]. Stainless chromium steel is resistant to NH4NO3 and (NH4)2SO4 solutions. Austenitic chromium and nickel steel are resistant to NH4NO3 and (NH4)2SO4 solutions. Zinc only exhibits resistance to corrosion in dilute solutions of ammonium chloride. Aluminum structures are resistant to attack by ammonium nitrate—which is a common ingredient in agricultural fertilizer.
Similar to other corrosive agents, sulphuric acid corrodes steel. The risk of corrosion is most pronounced in 60–100% acid solutions at low temperatures. Austenitic Cr-Ni steel can only be used at low concentrations or at high concentration and low temperature; corrosion. The rate of corrosion of about 1 mm/yr has been reported in 20–70% H2SO4 [2,7]. Zinc is severely corroded with increasing acid concentration and temperature.
2.3. Steel Structures
Steel is regarded as an alternative or complementary material to concrete. The factors that inform the selection of the material include the fact that it is less susceptible to corrosion and attack by acid, alkali, and other chemicals, as noted in the preceding section [2,7]. Steel is also resistant to attack by bacteria [8], and structures made of steel can be alloyed to form, nickel, chromium, and other forms of steel. Steel is also the material of choice in the construction of roofing materials for agricultural buildings [9]. Steel roofs are preferred because they are waterproof—a necessary requirement for long-lasting structures. The durability of steel structures can also be reinforced by hot-dipping or galvanizing. The treatment process for steel facilitates the formation of a robust bond between zinc atoms (on the surface) and the steel material. In addition, the treatment of steel ensures that the structure is covered with a uniform layer of base material to o er protection against the elements. According to the data in Figure 2, galvanized steel sheets have a probable lifetime of up to 70 years. However, only 10% of the material would achieve such high rates of survival. On average, galvanized steel roofs have a survival rate of 25–30 years [9].
Beyond galvanization and hot dipping, the durability of the steel structures is influenced by the frequency of exposure to the natural elements. Bertron notes that the risk of corrosion was exacerbated in steel (carbon steel) reinforcements in concrete that comes into contact with acids. In particular, exposure to acids in the presence of nitrate ions leads to the reduction of nitrate and the formation of ammonium ions. The reaction is optimized at a pH between 11 and 12. Therefore, the treatment of steel does not o er absolute protection against degradation.
3. Corrosion and Degradation of Structural Materials in Farm Environment
Farm structures are constructed using concrete, steel reinforcement, timber, and concrete substitutes [10–12]. However, the durability of concrete is the most pertinent factor; timber is used in limited applications because it is susceptible to fungal and insect attacks, discoloration, decay, swelling, and shrinkage [10]. Similarly, metallic structures are more susceptible to corrosion compared to concrete structures [2,11,13–15]. Concrete structures in farm environments are exposed to different forms of corrosive agents such as meal–water mixtures, acids, and bacteria, which suppress the pH and contribute to the accumulation of organic acids such as lactic acid, development of soluble calcium salts and leaching [12]. The cumulative effect is the degradation of the structural integrity of the material. However, the ease with which the concrete agricultural structures are degraded is dependent on the material properties of the concrete including water absorption, density, and compressive strength [12] The data in Table 4 shows the variation in material properties depending on the composition—cement content, gravel, water to cement ratio, sand, and other additions. Apart from the environmental factors, the durability of the structures is determined by metal reinforcements such as galvanized Z-girders, which are common in crop storage silos [11]. Beyond concrete and metal, the durability of timber support structures is dependent on the joint reinforcements, environmental factors such as exposure to harsh environmental conditions, and treatment [10].
3.1. Corrosion in Farm Environments
The corrosion of concrete and metallic structures is investigated from the perspective of microbial action on concrete, base, and acid-induced corrosion. The first sections explore the activity of fungi and bacteria on the corrosion of cement and metal structures.
3.2. Activity of Fungi and Bacteria
According to the information presented in the preceding sections, bacteria-induced corrosion has a negative effect on the durability of concrete structures. The risk of bacteria-induced corrosion is most pronounced if concrete structures are reinforced with steel, which in turn leads to the formation of FeS and FeP as the main products [16]. Emerging evidence has provided contrary evidence concerning the impact of bacteria on the corrosion of concrete structures.
Based on emerging evidence, bacteria and fungi have a mixed effect on concrete and metallic structures. The positive effects are linked to the presence of non-aggressive microbes, while the negative effects are linked to the proliferation of undesirable microorganisms [7,16]. The activity of bacteria and fungi in mediating corrosion is summarized in Figure 3 The image shows that heterotrophic bacteria and fungi operate optimally during mechanical and chemical corrosion. The process is dependent on the availability of sulfate salts (such as Gp, Anh, Bas, and Ett), silicate/carbonate aggregates, and cement (C-S-H-phases, Ca(OH)). In addition, the most active bacterial species in this phase include are Acidithiobacillus thiooxidans, Acidithiobacillus ferrooxidans, Thiobacillus intermedius, Halothiobacillus neopolitanus, Starkeya novella, and Thiobacillus tioparus [17] as shown in Figure 3; it is of note that corrosion cannot proceed unless the environmental conditions are appropriate—high relative humidity, average temperature, and higher concentrations of H2S and CO2. The durability of concrete and effects of acid-induced corrosion are discussed below.
3.3. Durability of Concrete and Acid-Induced Corrosion
Acid-induced corrosion involves the leakage of lactic and acetic acids onto the lime (pH ~13) in the concrete structures leading to the weakening as illustrated in Equations (1) and (2). The salts leached onto the concrete, leading to the weakening the binding agents and the cement paste, and consequently impacting the strength of the agricultural structure.
3.4. Acid Corrosion Mechanism in Cement Structures
3.4.1. Inorganic Acids
The underlying mechanism that dictates the rate of corrosion is dictated by the presence of H+ and OH ions. Nearly all types of cement are basic (pH > 12.5) due to the presence of Ca(OH)2. The high pH of the cement provides a protection against acid attack. The acid lowers the pH and destroys the concrete but the high pH does not exacerbate the attack. Exposure to organic (such as oxalic and acetic acid) or inorganic acids (H2SO4, HCl, H3PO4, and HNO3) leads to the lowering of the equilibrium and hydrolytic decomposition as shown in Equation 1 and 2, which impair the properties of the cement structures [18]. In particular, if the pH decreases below 12.5, the Portland cement dissolves [18]. A further decline in the pH leads to the dissolution of cement hydrates (such as calcium alumino-ferrite hydrates and calcium aluminate), which cannot withstand acid/neutral environments. The net effect is the formation of amorphous hydrogels, ferric oxides, Al2O3 aq, and Fe2O3 aq after complete degradation of the original cement microstructure [18]. The final acid attack products illustrate the adverse effects of acid attacks on the durability of concrete structures.
The severity of acid corrosion is influenced by time, as illustrated in Figure 4. The samples exposed to acid environments for 3–6 months (AC-3M and AC-6M) were highly corroded compared to those that were exposed for two months or the control (NC) [19]. The phenomenon illustrates there was a positive and definite relationship between the frequency of acid exposure and the durability of concrete structures [19]. Additionally, there were slight differences depending on whether the concrete specimens were exposed to biogenic (bio) or abiotic sulfuric acid; corrosion was most pronounced in the former case.
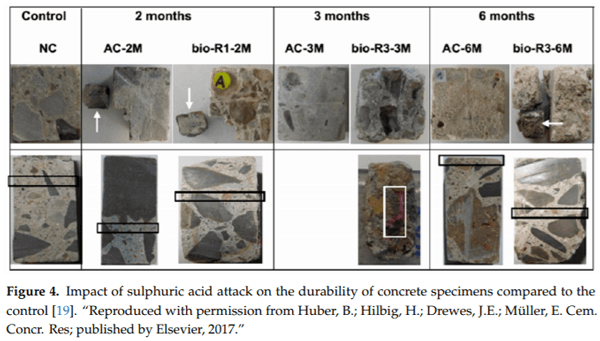
The corrosion of concrete and steel structures occurs in three primary steps. The first step is characterized by the diffusion of the effluents and other agricultural fluids that are found in farms. The fluids percolate through the pores in the concrete, weakening the structures [18]. The second step is characterized by an advanced chemical reaction between the fluids and the material. The physicochemical properties of the fluids influence the severity of the reaction. For example, acids aggressively attack cement through the mechanisms depicted in Equation 1 and Equation 2, leading to the formation of hydration products and the complete degradation of the concrete structure. The final step is characterized by the formation of soluble and insoluble products following advanced chemical reactions. Additionally, the insoluble products are deposited onto the corroded substances and the diffusion of the corroded items through the soluble layer.

Inorganic acids have a similar effect on the structural integrity of acidic structures. The impact of the acid attacks on the surface and cross-sectional morphology of the cement microstructure is visualized in the scanning electron micrographs in Figure 5a,b [15]. The decline in strength was proportional to the duration of exposure to acidic environments, as shown in Table 5. The compressive strength of the control (ordinary pozzolanic cement decreased from 29.4 MPa to 21.23 MPa after 90 days of exposure to nitric acid), as shown in Table 6. The data shows that mass losses increased consistently in acidic environments. Cement additive ratios (ultra-fine fly ash (UFFA) and fly ash (FA)) impact the mass losses. The least mass losses and reduction in compressive strength were reported in 50% FA + 10% UFFA—a phenomenon that underscores the importance of supplementary cementitious material on the durability of concrete structures exposed to acidic environments.
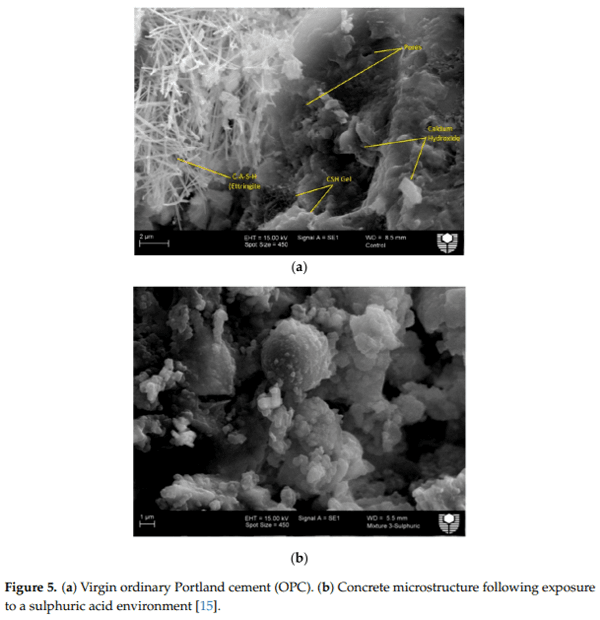
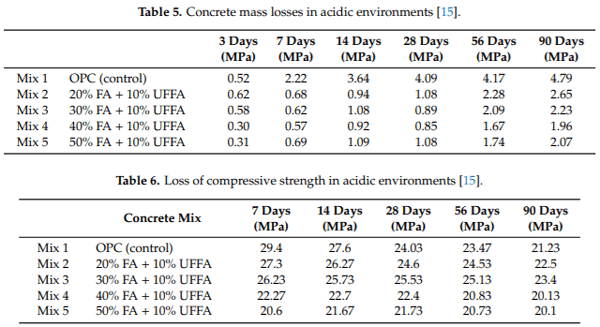
3.4.2. Organic Acids and Impact of Dissociation Constants
Organic acids induce corrosion and degradation of concrete using a similar approach as inorganic acids. Higher concentrations of acetic acid lead to the complexation of calcium in the cement leading to the formation of calcium and aluminum complexes. A summary of the reactions that define organic acid-induced corrosion of structures is presented in Table 7. In brief, it is of note that the formation of the complexes varies depending on the acid dissociation constant of organic acids. Oxalic acid has a lower dissociation constant compared to acetic acid pKa = 1.28 and 4.28 and 4.76, respectively [20]. Therefore, the risk of concrete degradation can be predicted using the acid dissociation constant. In general, weak and monoprotic acids pose a marginal risk to the durability of concrete structures compared to strong polyprotic acids.
3.4.3. Crop Storage Silos
Apart from structures that are in direct contact with manure, agricultural effluents, waste, and other liquids, the durability of the materials used in the construction of silos is impacted by heat and moisture [11]. Cleland noted that silos constructed using galvanized steel were corroded over time, even though stainless steel o ers long-term protection against corrosion. The corrosion was induced by the staining of the metal reinforcements by pulses, rapeseed, and wheat; it is of note that the process of corrosion progressed even though the produce was dehydrated to the required standards [11]. Beyond moisture, there were other factors that contributed to corrosion, including the fatty acids. Higher fatty acid concentrations translate to higher erucic acid. The rate of corrosion was most pronounced in mild steel compared to galvanic steel, as shown in Table 7 [11]. The observation is consistent with Safley and Westerman concerning the superiority of galvanized steel and chromium steel.
The produce stored in silos is susceptible to insect infestation and has to be treated by insecticides, which can increase the risk of corrosion. Alternatively, the constituent components in rapeseed, such as glucosinolates, triggered enzymatic activity leading to cellular disruption, loss of physical integrity leading to the release of nitriles, formation of acids and ammonia, and corrosion in general. Even though the type of products contributed to the risk of corrosion, optimal mass losses were reported in cases where there was optimal relative humidity, as shown in Table 8.
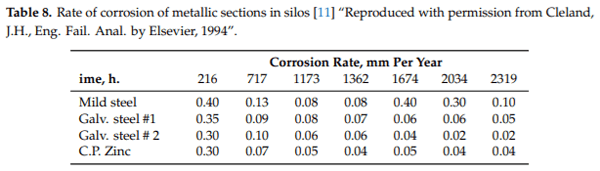
Supplementary explanation for Tables 7 and 8: The information in Table 7 show that the corrosion rate was higher in silos with 90% relative humidity and no oilseed rape. The least corrosion was reported at 51% relative humidity. The data in Table 8 show that mild steel is more susceptible to corrosion compared to galvanized steel. Galvanized steel #1 had as-cut edges while galvanized steel #2 had “had two coats of zinc-rich paint which overlapped the edges by 3.2 mm.”
4. Mitigation of Corrosion
Considering that corrosion has been identified as one of the threats to the structural integrity of steel structures, new materials have been developed to mitigate the risk of corrosion, including fiber-reinforced polymers [21], nanoscale pozzolanic and other cement additives [22], and stainless-clad reinforcing bars [23]. A greater emphasis is placed on fiber-reinforced polymers, which are among the most widely used materials in the mitigation of the risk of corrosion following the formation of a corrosion barrier.
Fiber-reinforced polymers are able to mitigate the risk of corrosion by limiting the rate at which water is absorbed and evaporated from the steel structures. Common Fibre-Reinforced Polymers (FRPs) include polyvinyl chloride (PVC), polystyrene (PS), polymethyl methacrylate (PMMA), polyisobutylene (PIB), polyamides, and polytetrafluorethylene (PTFE) [18]. In addition, the rate of dissolution, swelling, and precipitation is regulated in fiber-reinforced polymers—a phenomenon that is augmented by higher chemical resistance of the fiber polymers [18].
The rate of dissolution, swelling, and precipitation is regulated in fiber-reinforced polymers—a phenomenon that is augmented by higher chemical resistance of the fiber polymers [21]. The cumulative effect is greater stability of the concrete structure and reduced aging. Apart from limiting corrosion and aging of concrete structures, FRPs are preferred because they are non-toxic, have low life cycle costs, high usage, and resistance to fire and other natural hazards [24]. Thermal plastic and thermosetting FRPs also exhibit self-healing behaviors.
The mechanical properties (tensile and compressive strength) of the fiber-reinforced polymers are influenced by the fabrication process [25]. Kim and co-researchers have reported that the vacuum infusion fabrication method yielded FRPs with the best mechanical properties. Apart from the fabrication process, the qualities of the FRPs, mechanical and chemical properties are modified by additives such as antioxidants, light filters, and stabilizers, which mitigate oxidation and UV-damage. Even though FRPs undergo similar treatment, different materials have unique resistance to weak and strong acids and bases, as shown in Table 9. Based on the classification scheme, PVC is the ideal FRP for the protection of agricultural concrete structures.
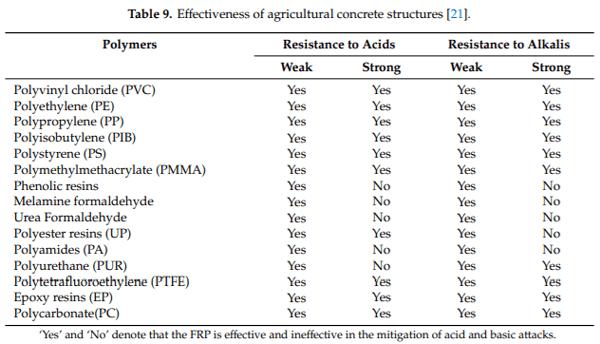
The properties of the fiber-reinforced polymers (resistance to weak and strong acid attacks) discussed above are influenced by the structural properties of the natural fibers. Empirical evidence suggests that fibers with higher concentrations of -cellulose exhibit greater resistance to corrosion due to their chemical stability and suitable mechanical properties, as shown in Table 10 [26]. In addition, cellulose-containing sisal fibers are easily available compared to pineapple. The chemical resistance of the sisal fibers is linked to the robust treatment process, which involves treatment with acetic acid and NaOH [26]. In particular, sisal has a moisture absorption property of 11%, elongation at failure of 2–3%, modulus of elasticity, and tensile strength of 38 and 600–700 MPa, respectively, as shown in Table 11. Following the comparison of the material properties of various fibers, flax has the most suitable material properties except for the percentage elongation at failure (1.2–1.6%) [24]. Additionally, the properties of FRPs are augmented by the incorporation of nanoparticles to improve the surface area. Therefore, the durability of FRPs in farm structures is influenced by additives such as nanoparticles, type of fiber, and polymer properties.
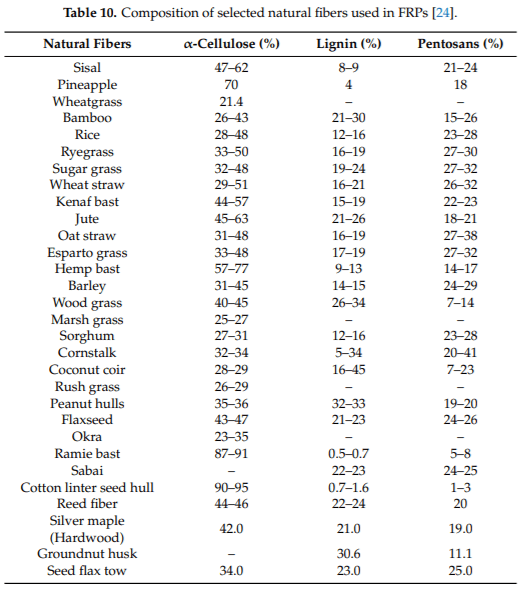
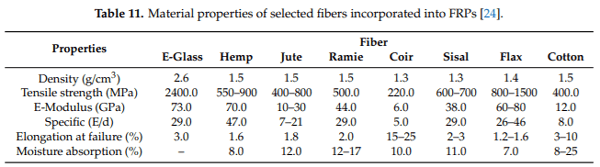
4.1. Sulfur Concrete
Apart from fiber-reinforced polymers and additives (such as fly ash), the risk of corrosion and environmental degradation of concrete in agricultural environments can be mitigated by sulfur concrete [27], which is modified form of concrete which uses sulfur as a binder. Material characterization experiments have shown the superiority of sulfur concrete compared to traditional Portland cement concrete—the superiority is evident from the physic-mechanical and chemical properties. In addition, sulfur has a strong binding ability for all elements except for noble gases [27]. Therefore, it has the ability to form robust bonds with concrete and prevent corrosion and degradation by aqueous solutions. On the downside, sulfur concrete is only widely used in North America. In addition, the material is susceptible to corrosion, considering that sulfur is soluble in non-polar solvents. In addition, the durability of sulfur is dependent on the type of allotrope used— there are at least 31 different S-allotropes [27]. According to research, the best S-allotrope for the formation of sulfur concrete is monoclinic because it is stable below 95 C, which is within the ambient temperatures in farm environments. However, the durability of sulfur concrete is also influenced by the addition of talc, alumina, fly ash, and micro silica, as shown in Figure 6. Sulfur concrete infused with alumina had the least apparent porosity (~1.3 to 1.5), which reduced the dissolution effect of HCl, H2SO4, and other corrosive agents [27].
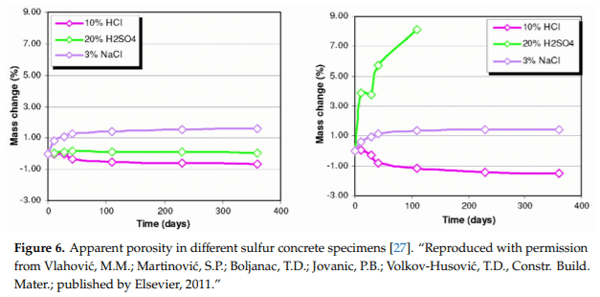
4.2. Fly Ash and Silica Fume Additives/Binders
The risk of corrosion and degradation of concrete structures in agricultural concrete can be mitigated by the integration of additives such as silica fumes and fly ash into traditional pozzolanic cement, as shown by Lapcharatsangroj and U-Tapao [28]. Concrete specimens labeled 10FA, 25FA, and 8SA22FA had variable mixes of supplementary cementing material (fly ash and silica fumes). The ratios of silica fume and fly have had a direct effect on the water demand, setting time, compressive strength and the average mass losses. The material characterization tests suggest that concrete sample 8SF22FA, which is made of 22% fly ash and 8% silica fumes. The superior performance of the cement sample with 8% silica fumes and 22% fly ash could be linked to the chemical composition of the binders, as shown in Table 12 [28]. Silica fumes (SF) contains twice as much SIO2 as fly ash (FA). In contrast, fly ash has slightly higher ratios of Fe2O3, Al2O3, K2O, and FeO3, which augment the strength of the concrete structure. The graphs in Figure 6a,b show that 8SF22FA had the highest compressive strength and least mass losses.
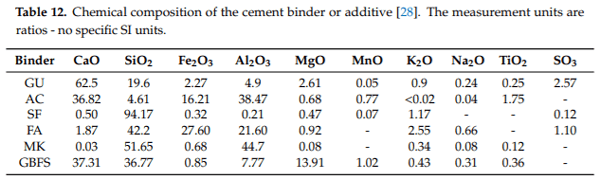
4.3. Design for Durability
The construction of agricultural structures, materials that o er optimal production against corrosion such as fly ash and silica fumes-infused concrete, does not provide the absolute guarantee for enhancing the durability of farm structures [29]. The observation is reinforced by the growing role of durability design—based on building codes and the incorporation of materials that satisfy the prerequisite requirements. For example, a research by Abdelmseeh and co-researchers showed that different concrete mixes (such as SSFC, SFC, PC40 (Portland cement concrete with a 0.4 W/CM ratio), and FASF) offered different levels of protection against hydrogen sulfide attacks after 8, 16, and 32 cycles. The least visual evidence of corrosion was reported in the SR (sulphate-resistant cement -CEM IIIB) concrete mix [30]. Beyond, the customization of the concrete structure application, the design for durability also encompasses the integration of adhesives and coatings and other surface modifications that are employed to improve the service life of the concrete structure.
Eurocode 2 (EN 1992-1-1 [35]) provided information for the durability design of concrete structures. Farm structures with high humidity are categorized as XC3 and with chemical attacks XA2 or XA3 depending to the conditions. For XC3, minimum concrete C35/45 and more than 15 mm of cover are required. For XA2 or XA3, special concrete composition is required according to Section 6 of EN 206-1 [36] together with the minimum required cover per EN 1992-1-1 [35].
Adhesives for Concrete
Various adhesives have been developed to augment the durability of the concrete structure, including epoxy-cement paste, which is made of epoxies such as bisphenol A and resins such as modified cycloaliphatic amine and modified aliphatic and aromatic amines [31,32]. The epoxy paste forms a robust bond with cement via silane surface functionalization (mechanical interlocking and hydrogen bonding); the net effect is better functionality of the material and resistance to moisture [32]. Similar observations were made by Yeong and co-researchers. However, the strength of the bond was influenced by the thickness of the epoxy, especially in the development of the shear strength of the concrete structure [31]. In addition, the effectiveness of the epoxy resin was also influenced by temperatures. Considering that the adhesives have limited thermal stability, elevated temperatures (>65 oC) diminish the mechanical strength of the epoxy resins by up to 90% [33]. The effectiveness of the adhesive was dependent on the ratios of the hardening agent and the resin, as shown in Figure 7. The thickness of the layers deposited varied between 1 mm and 3 mm. The epoxy resin with aromatic amines with a thickness of 4 mm exhibited the highest resistance against shear strain. In contrast, epoxy resins with a thickness of 7 mm exhibited the highest resistance against shear stress [34]. Apart from reinforcing bonds in concrete structures, adhesives are also used in steel structures using a similar mechanism as concrete applications.
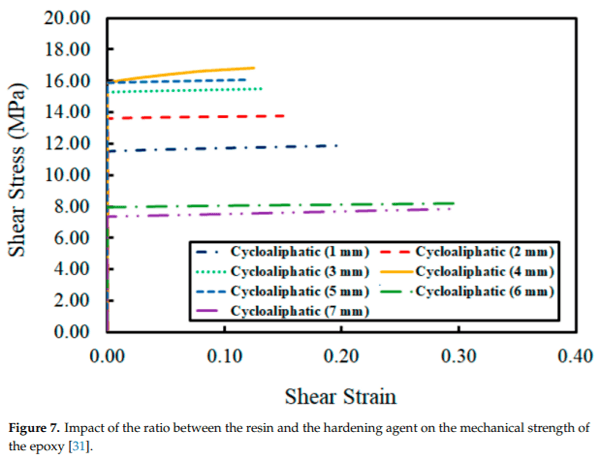
4.4. Repair and Rehabilitation
The repair and rehabilitation of concrete and steel farm structures is influenced by the projected service life of the building structure. The service life of a material employed in the construction of farm structures is influenced by the level of susceptibility to corrosion and degradation. Materials that have a higher risk of corrosion such as mild steel require constant repair and rehabilitation to extend their service life. In particular, concrete pipes used in farm environments are repaired using organic polymer binders, which are preferred because they do not require pipe excavation and do not induce shrinkage of the repaired structure [17]. The repaired section is protected from water by the application of water repellants such as silane or silane-siloxane or linings. On the downside, the sealants and binders might result in the accumulation of odorous and toxic substances such as H2S. Alternatively, the rehabilitation of concrete structures that are in direct contact with farm effluents can be achieved using geopolymer concretes, which are resistant to acid attacks, permeation, and abrasion. The ion exchange mechanism through which geo-polymers resist acid attacks is shown in Figure 8. The costs associated with repairs can also be mitigated by the infusion of bacteria-based self-healing materials [7], which autonomously expand and fill micro-cracks. The phenomenon reduces the need for further repairs.
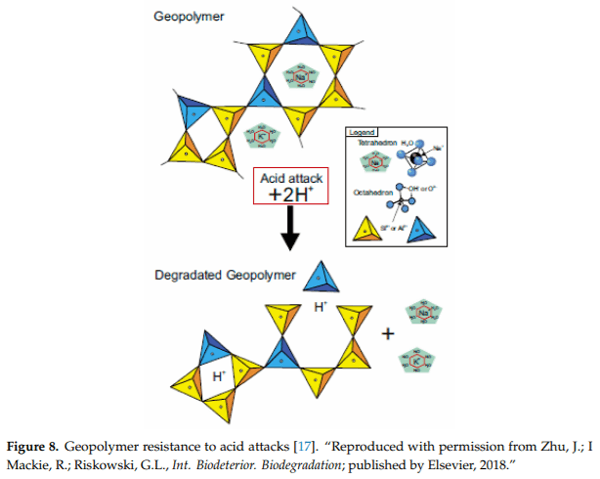
Especially for concrete, EN1504 [37] provides detailed information for the protection and repair of concrete structures (surface protection systems, structural and non-structural repair, concrete injection, chemical products like corrosion inhibitors, etc.).
For the corrosion protection of steel structures, EN ISO 12944-5:2019 [38] provides detailed information for the use of paint systems to prevent steel corrosion.
5. Conclusions
The review article explored durability issues and corrosion of structural materials and systems in the farm environment. Following the review of different publications relating to the corrosion of concrete and steel structures, the following observations were made. First, all materials (wood, metal, and concrete) used in the construction of farm structures are at risk of corrosion, which in turn compromises the durability of the structures and their service life. Metallic structures are easily corroded by chemicals found in animal manure products, organic and inorganic acids, leading to the formation of ferric and ferrous oxides and mass losses. Prolonged exposure of concrete structures to animal manure, agricultural effluents, and other chemicals results in the hydration of the cement, the formation of calcite crystals, and the disintegration of the structure. The threat to the durability of the structure is dependent on the composition of manure, and the nature of use—structures which are submerged in lagoons, or floor areas that come into contact with manure have a higher risk of corrosion compared to roofs and walls. In addition, poultry manure was associated with a pronounced risk of corrosion compared to swine or cattle manure, possibly due to the high concentration of H2S and NH3 and other corrosive agents. The durability of agricultural structures can be reinforced by the selection of corrosion-resistant materials such as chromium steel in place of BS, FRP concrete, and sulfur concrete in place of standard Portland concrete and concrete, which contains adequate ratios of fly ash and silica and nano additives. In brief, the degradation of agricultural structures cannot be avoided, but it can be partly mitigated in the material selection process.
This article was originally published in Applied Sciences. 2020, 10, 990; doi:10.3390/app10030990. This is an Open Access article distributed under the terms and conditions of the Creative Commons Attribution (CC BY) license (http://creativecommons.org/licenses/by/4.0/).